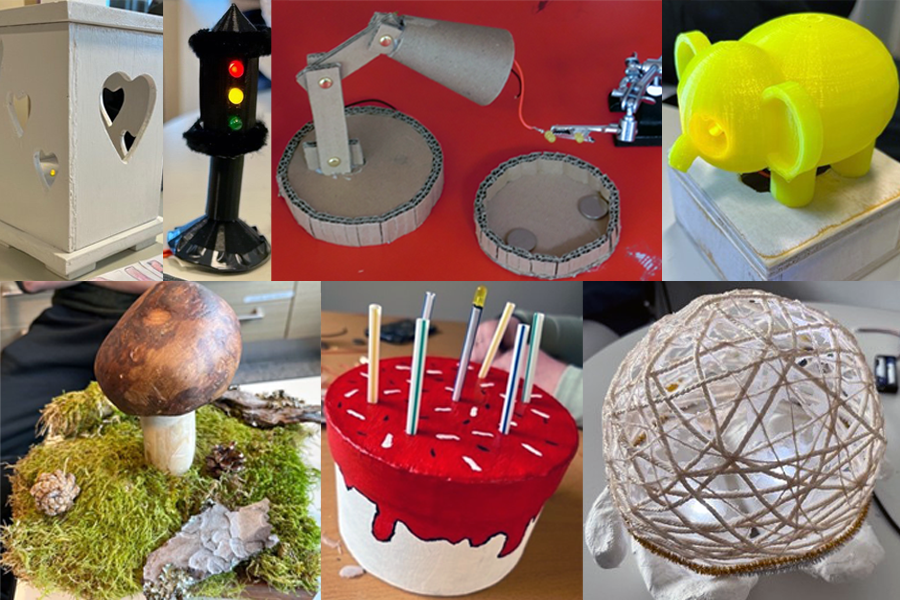
Design and build a smart lamp
Help students develop STEAM skills by building a smart lamp with this creative project that combines physics, programming, and art and…
From science fiction to reality: explore how continued innovation in 3D printing is supporting scientific progress in a range of different fields.
3D printing has been a fixture in science fiction since the late 1940s and 1950s. Perhaps the most iconic example is the replicator from Star Trek: The Next Generation (1987), a device that could synthesize anything from small food items to larger functional objects. At its core, the concept is both simple and ingenious: digital designs are transformed into physical, three-dimensional objects by adding material layer by layer or voxel by voxel (voxel=volume pixel) to create a final, potentially very complex, object without the need for prefabricated moulds. In contrast, subtractive manufacturing, for example, whittling or milling, creates an object by removing material from a large block of source material.
3D printing transitioned from science fiction to reality in the 1980s. However, for the first two decades, the technology remained largely confined to industrial settings due to the high cost of the machines. The open-source RepRap Project (a 3D printer, of which most parts could themselves be 3D printed) sparked the democratization of 3D printing, paving the way for widespread adoption in hobbyist, industrial, and research applications. Filament extrusion 3D printers like MakerBot’s Cupcake CNC led the charge, but today light-based 3D printers are just as accessible. Improvements in printing resolution and the variety of materials available have made 3D printing an invaluable tool for rapid prototyping, on-demand manufacturing, and research, offering versatility and innovation across various fields.
Filament 3D printers have become incredibly affordable over the last decade. Several companies sell reliable, well-supported machines for between 200€ and 500€, making them an accessible entry point into the hobby. Due to the growing popularity of 3D printing, many second-hand machines are available even cheaper. However, before purchasing a used printer, be sure to check the availability of replacement parts.
The internet offers access to numerous repositories, such as Thingiverse and Printables, which host free 3D assets for printing. The variety of available models is astonishing, ranging from small gadgets, toys, and tabletop role-playing miniatures to workshop accessories like a set of helping hands or tool organizers. You can even find detailed models of engines and anatomical joints or scans of dinosaur skeletons for educational purposes. If existing assets don’t meet your needs, 3D modelling software like Blender or FreeCAD allows you to create your own designs. Platforms like YouTube feature dozens of creators who provide video tutorials to teach the ins and outs of 3D modelling.
Of the various 3D printing technologies, two stand out as the most prominent: extrusion-based methods, such as fused deposition modelling (FDM), and light-based 3D printing. FDM works by heating a thermoplastic material, typically in the form of a filament, extruding it through a nozzle, and layering it to create a structure, with each layer fusing to the one below and next to it. This is the technology typically used by hobby-grade machines, and what most people picture when they think of 3D printing.
In contrast, light-based 3D printing uses a specific wavelength of light to harden selected areas of a liquid resin into a solid object. This technology allows very precise printing and is often found in academia and medical sectors where high-precision parts are required, for example, aligners in dentistry, but is also used for the fast generation of cheap prototypes or in the hobby sector to create miniatures.
In industrial settings, other forms of 3D printing are also commonly used, primarily a process called powder-bed fusion or selective laser sintering, with applications in aerospace, transportation, and industrial tooling. In this method, a powder – e.g., ceramic, polymer, or metal – is selectively heated with a laser, causing it to melt and form a solid object layer by layer. In the construction industry, extrusion-based printing processes use a type of cement, rather than polymer filament, allowing the 3D printing of entire houses.
This article mainly focuses on light-based 3D printing, the core technology employed in the Cluster of Excellence 3D Matter Made to Order (3DMM2O) located at Heidelberg University (UHD) and Karlsruhe Institute of Technology (KIT). Overall, there are various light-based 3D printing approaches that differ in execution, although they follow the same general principle: a photosensitive resin is hardened, a process typically referred to as ‘curing’, through exposure to a laser or an LED that triggers localized photo-polymerization to form the final 3D object.
What does ‘photo’ mean?
In this context, the prefix ‘photo’ refers to light. A photosensitive resin is a material that undergoes polymerization when exposed to light – a process known as photo-polymerization. This light can be visible, ultraviolet, or infrared, depending on the chosen printing technique. Although photosensitive resins often have optimal sensitivity to certain wavelengths, they may react to a broad range of wavelengths. For this reason, it is crucial to limit their exposure to white light, as it can prematurely trigger polymerization and compromise the 3D printing process. High-resolution 3D printers are typically housed in light-controlled environments that use yellow instead of white light to minimize such issues.
Different 3D printing methods offer distinct strengths and weaknesses. One important parameter is resolution. In the context of 3D printing, resolution refers to the smallest feature size that a printer can controllably create. FDM-based 3D printing is known for its speed and is primarily used to create larger objects, with typical resolutions of around 100 microns. In contrast, light-based 3D printing methods excel in high resolutions and offer a highly defined surface texture, but are generally slower and better suited for producing smaller, more detailed objects.
Commercial 3D printing solutions offer a wide range of resolutions and materials, but they face significant limitations at the micro, nano, and molecular scales. For example, most state-of-the-art commercial light-based 3D printers can achieve a maximum resolution of only 10 microns. Moving 3D printing towards the nanoscale by using technologically advanced light-based microprinters opens new possibilities for researchers in a wide variety of fields, from the study of cellular interactions to printed electronics and microrobotics. However, to truly reach the next generation of 3D printing technology, advancements in three key areas are required: the digital designs or models to be printed, the materials used for the printing process, and the printers themselves. Progress in these areas opens the doors to many innovative applications for 3D-printed microstructures in electronics, optics, computing, and the life sciences.
Many research groups all over the world work on advancing all four areas of 3D printing. Here, we focus on research conducted by the Cluster of Excellence 3DMM2O. Guided by the slogan “finer, faster, and more,” 3DMM2O researchers aim to improve spatial resolution, enhance manufacturing scalability, and expand the variety of printable materials.[1] Additionally, they explore ground-breaking applications in engineering and the life sciences, particularly at the nanometre scale. Below, we present two recent applications of 3D printing in research and explore how microalgae can help make 3D printing more sustainable.
Studying how confinement and 3D environments affect cells is a key area of interest in cancer research. One approach to investigating these conditions involves using a model system, specifically spherical aggregates of a few hundred cells known as spheroids. Spheroids can mimic the tissue and behaviour of tumours in an environment in a tightly controlled environment, which makes them a potent model for cancer research and drug testing. Traditionally, these spheroids are analyzed while floating in miniaturized flasks. However, researchers have developed an innovative, scalable method to confine the spheroids by 3D printing an igloo-like structure with nanometre resolution around them, without compromising their viability.[2] This simplifies the processing of large numbers of spheroids and enables high-throughput experimentation, that is, experimental setups that can produce a lot of data in a short time with comparatively little human input, usually by investigating different variables in parallel. Consequently, researchers can conduct more tests, explore a broader range of conditions, and accelerate progress in personalized medicine.
When a photosensitive resin cures, its molecules form covalent bonds. These bonds are exceptionally strong, but lack adaptability and reprocessability, leading to rigid 3D structures that cannot be functionalized or easily recycled, which raises significant environmental concerns. To overcome this challenge, researchers have developed novel resins that form dynamic covalent bonds during curing.[3] These bonds can break and reform in response to external stimuli, such as heat, light, or changes in pH. Consequently, the printed objects exhibit exceptional properties, including the ability to change shape and size, self-heal, and be recycled.
3D printing is inherently more sustainable than subtractive methods, primarily because it generates significantly less waste. However, the process still has an environmental impact because it relies on thermoplastics (extrusion-based 3D printers) or photoresins (light-based 3D printers) typically derived from petrochemicals. This dependency contributes to the depletion of fossil fuels and the release of greenhouse gases.
To improve sustainability, researchers are exploring microalgae oil as an alternative and renewable source of photoresins.[5] A standard photoresin contains two key components: the photoreactive monomers and a photoinitiator. When exposed to light, the photoinitiator becomes excited and transfers energy to the photoreactive monomers, thereby triggering polymerization, a chain reaction of monomers bonding with each other to form a solid structure.
The researchers took advantage of the naturally high lipid content of microalgae – primarily triglycerides – by functionalizing them to serve as the photoreactive monomers. Meanwhile, the algae’s native chlorophyll acts as the photoinitiator. These bio-inks enable the creation of 3D structures with sub-micron resolution, low toxicity, and biocompatibility, making them suitable materials for applications in biological research or potentially even medicine. They also originate from a regenerative, sustainable resource.
3D printing has evolved from a science-fiction concept into a transformative technology driving numerous scientific advancements. While we are still far away from achieving the futuristic visions depicted in sci-fi stories, researchers are working to bridge the gap between imagination and reality. We hope this article has offered an engaging glimpse into the world of 3D printing, highlighting not only the technology itself but also the groundbreaking work of 3DMM2O and other researchers who are expanding its possibilities and pushing its limits.
Bio-ink: Here, bio-ink refers to inks (photoresins) derived from living biological sources, such as microalgae.
Biocompatibility: Compatibility with biological systems. Some materials are not biocompatible; they may be toxic to or interfere with biological processes.
Extrusion-based 3D printer: A 3D printer that deposits material layer by layer, typically fusing adjacent layers together to form a solid 3D object.
Light-based 3D printer: A 3D printer that selectively cures a photosensitive resin by using light, layer by layer, to create a solid 3D object.
Microstructure: The structure of an object at the micrometre level, which can differ significantly from its appearance at the macroscopic scale (visible to the naked eye).
Minimum feature size: The smallest possible detail that a 3D printer can reliably produce.
Nanoscale: A nanoscale object has sub-micrometre features, typically ranging from 1 to 1000 nanometres in size.
Photoresin: A photoresin consists of monomers and photoinitiator molecules. When exposed to light of a specific wavelength, the photoinitiators trigger a chain reaction that converts monomers into polymers, causing the resin to solidify.
Polymer: A polymer is a macromolecule consisting of many repeating subunits.
Resolution: In 3D printing, resolution refers to the minimum distance between two printed objects (typically lines in a test) at which these objects can still be distinguished. The smaller this distance, the better the resolution and the higher the possible degree of detail.
Scalability: In experiments or manufacturing, scalability refers to how well a process can be adapted for high-throughput production or mass manufacturing. Achieving scalability often requires significant modifications to the setup or the use of specialized tools.
Thermoplastic material: A thermoplastic material softens when heated to a certain temperature and solidifies upon cooling. Unlike materials that char when heated, thermoplastics can be reshaped multiple times. Voxel: A pixel is a discrete point in an XY-coordinate system. A volume pixel, or voxel, adds a third dimension (Z axis) to the coordinate system. In high-resolution 3D printing, a voxel represents the smallest possible 3D unit that a printer can produce.
Voxel: A pixel is a discrete point in an XY-coordinate system. A volume pixel, or voxel, adds a third dimension (Z axis) to the coordinate system. In high-resolution 3D printing, a voxel represents the smallest possible 3D unit that a printer can produce.
[1] Blasco E et al. (2024) 3D Matter Made to Order. Advanced Functional Material 34. doi: 10.1002/adfm.202315919
[2] Taale M et al. (2024) In Situ Fabrication of Constraints for Multicellular Micro-Spheroids Using Two-Photon Lithography. Advanced Functional Material 34. doi: 10.1002/adfm.202302356
[3] Zhu G et al. (2024) Introducing Dynamic Bonds in Light-based 3D Printing. Advanced Functional Material 34. doi: 10.1002/adfm.202300456
[4] Jia Y et al. (2022) Covalent Adaptable Microstructures via Combining Two-Photon Laser Printing and Alkoxyamine Chemistry: Toward Living 3D Microstructures. Advanced Functional Material 33. doi: 10.1002/adfm.202207826
[5] Vazquez-Martel C et al. (2024) Printing Green: Microalgae-Based Materials for 3D Printing with Light. Advanced Functional Material 36. doi: 10.1002/adma.202402786
This article offers a fascinating exploration of 3D printing, from its origins in science fiction to its transformative applications in research and technology today. Teachers can use it as a springboard for interdisciplinary lessons, linking science, technology, engineering, art, and even ethics. While the article delves into complex topics like photopolymerization and high-resolution printing, it provides a valuable opportunity to introduce these concepts in an accessible way. For educators, it presents a chance to engage students with real-world examples, such as cancer research and sustainable materials, while also prompting discussions on the environmental and societal impacts of emerging technologies.
To make the article even more relevant for high school students, consider integrating local examples of 3D printing applications, or pairing the article with hands-on projects. Students could explore simple 3D modeling software, design basic objects, or research how 3D printing is being used in their communities. By adding these practical components, teachers can help bridge the gap between theory and application, making the topic of 3D printing both engaging and tangible for learners across various subjects.
Annamaria Lisotti, Liceo STEAM International Emilia, Italy
Help students develop STEAM skills by building a smart lamp with this creative project that combines physics, programming, and art and…
Is it a solid? Is it a liquid? It’s slime! Make slime to explore viscoelasticity and then complete a material science design challenge.
Basic research is often misunderstood by the public and misconstrued by the media. Try this role play to learn how research is funded and how basic…