Fusion in the Universe: where your jewellery comes from Understand article
Does alchemy sound too good to be true? Paola Rebusco, Henri Boffin and Douglas Pierce-Price, from ESO in Garching, Germany, describe how creating gold – and other heavy metals – is possible, though sadly not in the laboratory.
How are heavy elements formed? The last episode of the ‘Fusion in the Universe’ saga (Boffin & Pierce-Price, 2007) ended with the production of iron, but the nucleosynthesis adventure – in which atomic nuclei are created – does not stop there. Let us refresh our memory. In the first few minutes after the Big Bang, the temperature of the newborn Universe cooled down (to a few billion degrees!) to form hydrogen and helium. Stars spend most of their life burning hydrogen into helium. Only when temperature and pressure become high enough do they start to fuse helium atoms, forming new elements. Lighter elements are the bricks that successively fuse together to produce heavier elements, up to iron-56.
Iron-56 has the most stable nucleus because it has the maximum nuclear binding energy (see box and diagram below). Nature cherishes stable configurations and therefore the fusion process described in our last article, which brings us from hydrogen up to heavier, more stable nuclei, will not continue beyond iron-56. So, where do heavier elements such as lead, silver, gold and uranium come from? There is no magic: the Universe provides other fascinating ways to produce all the heavy elements. In the high temperature and pressure of a star, fusion is as spontaneous as rolling down a hill (a process that releases energy). However, these new mechanisms are more laborious, like climbing a hill (a process that needs energy). Furthermore the next stages of nucleosynthesis are quite hectic, as they involve captures and explosions. Three types of capture are involved, two dealing with the capture of neutrons (the s- and r-processes) and one with the capture of protons (the p-process).
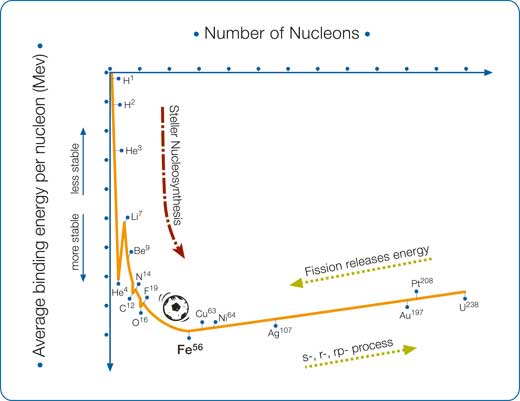
Image courtesy of Mafalda Martins, ESO
Neutron capture
One route to create elements heavier than iron-56 starts when extra neutrons collide and fuse with an existing nucleus. In this way we get neutron-richer, heavier nuclei, but with the same number of protons, or the same atomic number. These nuclei are just heavier isotopes of the original element, so we have not yet achieved our aim of creating a heavier, different element.
However, the process has not yet finished. These new isotopes may be stable or unstable, depending on their number of protons and neutrons. If the neutron capture produces an unstable isotope, then it can undergo a spontaneous radioactive decay. One such decay is ‘beta decay’, in which an electron and an anti-neutrino are emitted, so that one of the nucleus’ neutrons is converted into a proton. The net result of this conversion is a nucleus with one more proton and one fewer neutrons. Since the number of protons has changed, this has indeed produced a new, different element.
In this process of neutron capture followed by beta decay, it is important whether the initial neutron capture is slow or rapid relative to the beta decay. The two cases, referred to respectively as the s-process and r-process, produce different elements and occur in different circumstances in the Universe.
Slow neutron capture: the s-process
Each neutron capture in the s-process converts a nucleus to an isotope of the same element with one more neutron. Eventually, these single increases in neutron number lead to an unstable isotope. Because the neutron capture is relatively slow in the s-process, the unstable nucleus beta-decays before any more neutrons can be captured. In other words, as soon as the first unstable configuration is reached, a beta decay turns the nucleus into one with one more proton and one fewer neutron (see diagram below).
Where in the Universe can we find the right conditions for the s-process to occur? It turns out that it can occur during the late stages of the life of Sun-like stars. We already know (see, for example, Boffin & Pierce-Price 2007) that if the initial mass of a star is comparable to that of the Sun, then at the end of the star’s life, it runs out of fuel and cools to become a white dwarf. Before it cools down, free neutrons are produced (mainly from the decay of carbon and neon): they are plentiful enough to produce heavy elements via slow neutron capture. In this way, elements such as barium, copper, osmium, strontium and technetium are produced.
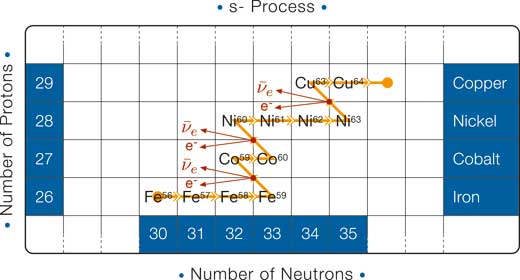
Note that the horizontal track in the s-process is shorter than in the r-process (in the s-process fewer neutrons are captured); as a consequence the movement in the vertical direction is also shorter (there are fewer neutrons that can be converted into protons).
Image courtesy of Mafalda Martins, ESO
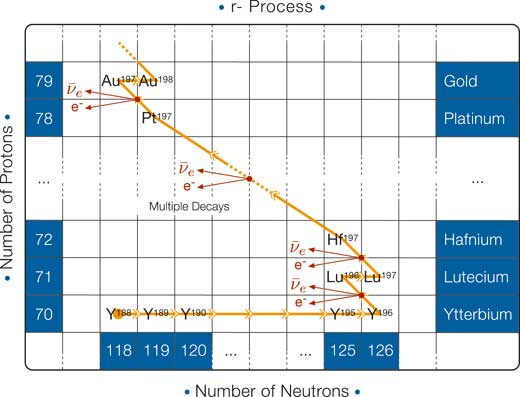
Image courtesy of Mafalda Martins, ESO
Rapid neutron capture: the r-process
If, instead, the neutrons are produced at a very high rate, then the unstable nuclei that are formed have enough time to swallow many neutrons that subsequently decay in cascade into protons (see diagram above): this is how the elements with the highest atomic number are synthesised in nature.
Let us discover where the r-process takes place in the Universe. As was also discussed in the previous article, when the mass of a star is greater than about eight solar masses, the temperature and pressure at its centre become high enough to trigger the fusion of carbon and oxygen and, ultimately, to form a core of iron. In this final stage, a star’s interior is very like an onion (see diagram below): the outermost envelope is composed of hydrogen and helium, with the inner layers consisting of progressively heavier nuclei, due to successive fusion reactions.
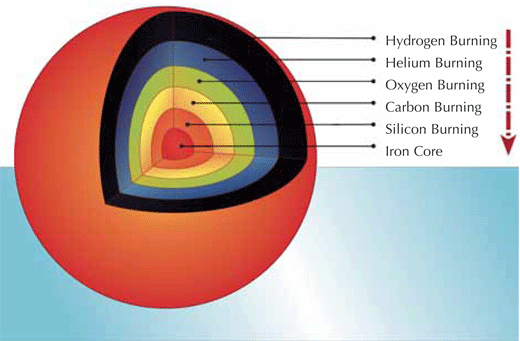
Image courtesy of Mafalda Martins, ESO
Iron is too stable to start to burn, hence it accumulates and the iron core continues to increase. There is, however, a mass limit (called the Chandrasekhar limit) above which the iron core can no longer grow, as its gravity becomes too high for it to support itself. At this point a catastrophic collapse (with the outer layers of the core reaching velocities up to 250 million km/h) shrinks the core, until the infalling matter bounces back and all the energy is transferred to the outer layers, in a titanic explosion (see below). This phenomenon is called a supernova explosion, specifically a Type II supernova (SN II).
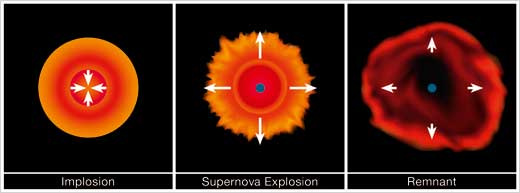
Image courtesy of Mafalda Martins, ESO
It is in the collapsing iron core of SN II that the r-process occurs. During collapse, electrons and protons merge to produce neutrons and neutrinos. The flux (the number per unit time and unit area) of neutrons is so high (of the order of 1022 neutrons per cm2/s) that a nucleus has time to capture many neutrons before it beta-decays. Gold, europium, lanthanum, polonium, thorium and uranium are some of the elements produced through the r-process.
Proton capture
Another process by which heavier nuclei are produced is via proton capture (p-process). However, a large nucleus containing many protons has a high positive charge, which repels additional approaching protons. This repulsion (the Coulomb barrier) is very high, and ensures that proton capture is a much rarer event than neutron capture. To be absorbed by the nucleus, a free proton must be very energetic, so this process only takes place at very high temperatures.
So where can we find high enough temperatures for proton capture? Again, we look to the stars. Although our own Solar System has only one star – the Sun – a large number of stars are actually in systems with at least two stars. When two stars are orbiting each other, they form a ‘binary system’. If the stars are close enough, it is possible for one star with a strong gravitational pull to ‘steal’ gas from its companion star. This can happen, for example, when a massive, compact white dwarf or neutron star pulls hydrogen-rich gas down onto its surface from its partner. This material provides a flow of free protons, hot and energetic enough to overcome the Coulomb barrier and fuse with other nuclei. Lanthanum, ruthenium and samarium are typical elements produced in the p-process.
Conclusion
We have seen how, although nuclear fusion in stars produces elements only up to iron-56, heavier elements are produced by a variety of processes. These nucleosynthesis processes, involving the capture of neutrons or protons, and radioactive decays, happen in exotic situations in the Universe. Slow neutron capture can occur late in the lives of Sun-like stars, before they end their days as white dwarfs. Proton capture is a result of a white dwarf or neutron star cannibalising gas from an unfortunate companion star. And rapid neutron capture takes place during the catastrophic stellar collapse which occurs just before the dramatic explosion of a Type II supernova. By changing one element into another, these fascinating natural processes achieve what mediaeval alchemists could not – the transformation of base metals into (among other elements) gold.
Nevertheless, we cannot blame the alchemists. Their laboratories may have been well equipped, but they lacked a key piece of apparatus: a supernova explosion.
The mystery of the vanished mass
The nuclear binding energy is the amount of energy needed to break a nucleus apart into protons and neutrons. It is also the energy that two particles release when they merge. Let’s imagine you have a proton and a neutron and that they have the same mass (a very good approximation). Push them together until they merge and they will form a deuterium nucleus. What is its mass? If the proton has mass 1 and the neutron has mass 1, you would expect 2, wouldn’t you? Not so: the mass of a deuterium nucleus is lower than the sum of the two – some mass has vanished! The solution lies in the famous Einstein equation, E = mc2. When two particles merge, they release the nuclear binding energy EB, but since energy and mass are equivalent, this means that the correspondent mass, mB= EB/c2, is lost.
Let’s consider first helium-4 and then iron-56. In atomic mass units (u = 1.66 x 10-27 kg = 931.5 MeV/c2) the mass of a proton and a neutron are mP = 1.00728 u and mn = 1.00866 u, respectively. The measured mass of a nucleus of helium-4 is mHe = 4.00150 u, while the sum of the mass of its components is 2mP + 2mn = 4.03188. The difference gives the mass 4.03188 u – 4.00150 u = 0.03038 u, which corresponds to a total binding energy of approximately 28.3 MeV (the binding energy per nucleon is 28.3/(2 + 2) = 7.07 MeV).
If you repeat the same steps for iron-56 (which consists of 26 protons and 30 neutrons), the total binding energy is much greater: about 492.2 MeV, or 8.79 MeV per nucleon. This extreme stability places iron-56 at the lowest point of the curve in the binding energy plot, and fusion to heavier elements would be an ‘uphill’ process, requiring the input of energy. This is why, although helium-4 nuclei can be readily fused into heavier elements, more extreme processes (described in this article) are required to obtain elements heavier than iron-56.
Margaret Burbidge and the B2HF team
The mechanisms behind the production of the heavier elements (the s- and r-processes) were first pointed out in a long theoretical paper published in 1957: ‘Synthesis of the elements in stars’ (Burbidge et al., 1957). This revolutionary and still up-to-date paper is signed B2HF – not a strange chemical compound but the initials of the surnames of the scientists who wrote it: Margaret Burbidge, Geoffrey Burbidge, William Fowler and Fred Hoyle.
The British astronomer Margaret Burbidge was born in 1919 and is still active in research, as professor emeritus of physics at the University of California, San Diego, USA. When she was a teenager, her grandfather gave her popular books on astronomy: “I saw my fascination with the stars, born at age 4”, she writes in her witty autobiography (Burbidge, 1994), “linked with my other delight, large numbers.” Her life has been full of scientific discoveries and political fights; it was not always easy to be a female scientist but she never gave up. “If you meet with a blockage, find a way around it,” she suggests. The rest of the group is no less notable: Fred Hoyle and Margaret’s husband, Geoffrey Burbidge, are most famous for their iconoclastic theories opposing the Big Bang theory, while William Fowler shared the 1983 Nobel Prize in Physics for his theoretical and experimental studies on nucleosynthesis.
References
- Boffin H, Pierce-Price D (2007) Fusion in the Universe: we are all stardust. Science in School 4: 61-63.
- Burbidge EM, Burbidge GR, Fowler WA, Hoyle F (1957) Synthesis of the elements in stars. Reviews of Modern Physics 29: 547-650
- Burbidge EM (1994) Watcher of the skies. Annual Review of Astronomy and Astrophysics 32: 1-36
Resources
- To discover when and where the latest supernovae have detonated, see the Supernovae website, where scientists and amateurs hunt and register new supernova explosions: www.supernovae.net