Fusion in the Universe: we are all stardust Understand article
Henri Boffin and Douglas Pierce-Price from ESO, in Garching, Germany, investigate our celestial ancestry.
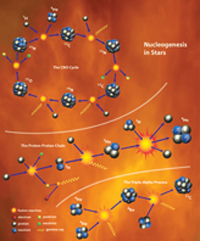
Image courtesy of Mark Tiele
Westra
The next time you gaze at stars during an evening stroll, you should spare some companionable thoughts for these glowing balls wandering silently in the vast Universe. For you are looking, in a sense, at your ancestors: humans, all other living creatures on Earth – and Earth itself – are children of the stars! Most of the elements of which we are made or which allow us to live – carbon, oxygen, nitrogen and many others – were created in stars. Even the fluorine that makes our teeth strong!
Matter as we know it is composed of three-quarters hydrogen and roughly one-quarter helium. All the other elements come only in tiny amounts, and astronomers tend to call these trace elements simply ‘metals’, even if the elements are not really metallic. The hydrogen and helium were created right at the start, in the very first minutes of the existence of the Universe. Less than one second after the Big Bang, the event through which everything came into being, fleeting energetic particles were ‘frozen’ into the future components of atomic nuclei: protons and neutrons. The ‘freezing’ was very relative, however, as the temperature at that instant was still several hundreds of billions of degrees!
During the first second after the Big Bang, the temperature was so high that protons and neutrons were in equilibrium. Soon, however, the temperature dropped below a critical value, and neutrons started to decay, each producing a proton, an electron and an anti-neutrino. This decay was possible because neutrons have a higher mass than protons. The Universe would quickly have become devoid of neutrons were it not for a reaction that ‘preserved’ them, by combining a neutron and a proton to form a deuteron, the nucleus of deuterium.
Deuterium is also called heavy hydrogen and is most famous for its importance in the nuclear bombs that the Germans tried to make during World War II. Once deuterons have formed, other heavier nuclei can form. When two deuterons collide, they produce a neutron and a helium nucleus – in its light helium-3 variant, consisting of one neutron and two protons. The process may then continue one step further, producing the more common helium-4 nucleus, consisting of two neutrons and two protons. Almost all the neutrons in the Universe end up in normal helium-4 nuclei, but a few helium nuclei combine into heavier nuclei, giving a small amount of lithium-7.
So, three parts hydrogen, one part helium-4, a little deuterium and helium-3, and a pinch of lithium. A few minutes after the Big Bang, the great cosmic soup has been prepared, the basis of all the other ingredients yet to come in the Universe: stars, planets and, eventually, life. Amazingly, the preparation of this soup is so simple that even the least experienced cook could try it. In fact, the balance of ingredients depends on a single parameter: the initial density of protons and neutrons. When astronomers measured the amount of these elements in the Universe, they found values that agreed very well with those predicted by the theory. This close agreement was one of the nails in the coffin of opponents to the Big Bang theory.
But what about the heavier elements? If they were not created in the first moments of the Universe, where do they come from and when were they created? The answer lies in the stars.
In the interiors of stars, temperature and density are high enough to overcome the forces that cause atomic nuclei to repel each other, allowing them, instead, to fuse. In Issue 3 of Science in School, we saw that the power of the Sun comes from the fusion of hydrogen nuclei to form helium in its inner core (Westra, 2006). The same happens in all stars that are in the ‘main sequence’, the stage at which they burn hydrogen.
The mechanism by which stars produce helium from hydrogen depends on the mass of the star: stars of the same or smaller mass than the Sun convert hydrogen into helium mostly through the ‘proton-proton chain’ (see image). In more massive stars, the main mechanism is the ‘CNO cycle’, in which carbon, nitrogen and oxygen atoms act as catalysts for the fusion of hydrogen into helium (see image).
The CNO cycle raises an apparent paradox: if the carbon, nitrogen and oxygen elements are themselves produced in stars, how can they be used as catalysts to fuse hydrogen into helium? This solution lies in the fact that stars form from the remains of previous generations of stars. The very first stars did indeed contain only hydrogen and helium, which they converted into heavier elements. These heavier elements were liberated into the interstellar medium when the stars exploded as supernovae. The interstellar medium became progressively enriched in carbon, nitrogen and oxygen, and the next generation of stars formed with a small amount of these elements, enough to act as catalysts.
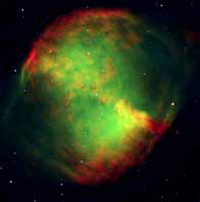
consisting of very rarefied
gas that has been ejected
from the hot central star
(visible on this image), in one
of its last evolutionary stages
Image courtesy of ESO
During the main sequence, the longest period in the life of a star, hydrogen is thus converted into helium. Eventually, hydrogen is depleted in the centre of the star, where burning occurs, and the star evolves, becoming larger, cooler, and redder – a ‘red giant’. After a brief phase, the temperature and density in the core increase sufficiently for new reactions to occur. This time, it is helium that starts to burn. Two helium nuclei can fuse to form a beryllium nucleus. Although beryllium nuclei are unstable, and most will rapidly disintegrate, some will collide with another helium nucleus, forming carbon. The net result is thus that three helium nuclei form a carbon nucleus. A fraction of the carbon nuclei thus formed collide with further helium nuclei to form oxygen. In the cores of these giant stars, therefore, helium is converted to a mixture of carbon and oxygen.
For stars only a few times bigger than our Sun, this will be the end. Once this carbon-oxygen core has formed, the star ejects its outer envelope in the form of a ‘planetary nebula’, leaving behind a white dwarf.
For more massive stars, however, the adventure continues. The gravitational force exerted by such a star is still strong, causing the core to contract further, thereby increasing the density and the temperature so that further nuclear reactions, leading to yet heavier elements, are possible. In this way, elements such as neon, magnesium and silicon, and later sulphur, chlorine and calcium are produced. All these elements have a number of nucleons that is a multiple of four, as they come from the combination of helium nuclei. As the helium nucleus is also called an alpha-particle, these elements are known as alpha-elements and are more abundant than other heavier elements.
However, helium capture is not the only possible process by which heavier elements are formed. Nuclei can also, more rarely, capture other particles, such as neutrons, protons and deuterons. In this way, a variety of elements, such as fluorine or sodium, can be produced. These elements, however, are present in smaller quantities.
Finally, nickel-56 can be formed in the alpha-process (by the combination of helium nuclei). This nucleus – with 28 protons and 28 neutrons – is unstable, and spontaneously decays into iron-56, which has a stable 26 protons and 30 neutrons. Up to this point, all the reactions that have taken place in the star have produced energy, which allowed the star to continue its life and ‘fight’ against gravity. But with the formation of iron-56, this is no longer possible. Being the most stable nucleus that exists – it has the highest nuclear binding energy – iron-56 can only be transformed into other elements by putting energy in, rather than getting energy out. The star cannot use these nuclear reactions to support itself. The production of a core of iron in a massive star is thus a prophecy of doom: the star can no longer fight against gravity. It collapses, and the ensuing rebound and shock wave end its life in a grandiose and dramatic explosion: a supernova. By exploding, the star will cast into the interstellar medium all the elements it has created – and others it will create just before dying. But that is another story….
A genius’ prediction
The ‘triple-alpha process’ is an example of helium capture. It is a two-step reaction in which a carbon nucleus is formed from three helium nuclei. First, two alpha-particles (helium nuclei) collide to form a beryllium-8 nucleus. This is unstable and decays very rapidly. On the face of it, it is unlikely that a third helium nucleus could be captured before the beryllium-8 decays. To create significant amounts of carbon in the Universe, some additional factor which makes a successful combination more likely would be needed.
But, we know that carbon is created – if it were not, then we humans, and all life on Earth, would not be here to discuss it, and you would not be reading this article! Using this simple but profound argument, the famous British astronomer Fred Hoyle (1915-2001), in a characteristic stroke of genius, predicted that some additional helping factor must indeed exist. Scientists turned to laboratory experiments and, sure enough, discovered a previously unknown ‘resonance’, a matching of energy levels between the beryllium-8 and helium-4 nuclei, and the carbon-12 nucleus that they form. This resonance greatly increases the probability of a successful combination, just as Hoyle predicted. This is a fascinating example of a scientific prediction being made from an argument based on the simple fact that scientists (and life itself) even exist to think about it.
References
- Westra MT (2006) Fusion in the Universe: the power of the Sun. Science in School 3: 60-62.
Web References
- For more information about ESO (the European Organisation for Astronomical Research in the Southern Hemisphere) and its educational projects, visit: www.eso.org/public/outreach/eduoff/