Spinal cord injury: do stem cells have the answer? Understand article
Spinal cord injury typically causes permanent paralysis and is currently a condition without a cure. Could stem cell therapy provide hope?
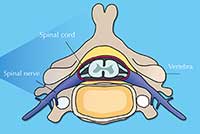
Wikimedia Commons
American actor and activist Christopher Reeve will be remembered for his leading role in the 1978 blockbuster movie Superman. Sadly, he will also be remembered as a man whose tremendously active life, both on and off screen, was shattered by a catastrophic injury that left him paralysed from the neck downwards – a state in which he remained until he died in 2004.
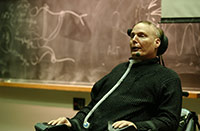
the potential benefits of
stem cell research at a
neuroscienceconference at
the Massachusetts Institute
of Technology, USA, in 2003.
Image courtesy of Mike Lin /
Wikimedia Commons
In May 1995, during an equestrian competition, Reeve was thrown headfirst off his horse. The weight of his body was thrust through his spine, breaking two of the vertebrae in his neck and causing extensive damage to his spinal cordw1.
What happened during his accident – at the level of blood, bones, cells and molecules – to cause his life-long paralysis? And how might research into new treatments based on stem cells offer hope for people paralysed by spinal cord injury? Could it help them to regain some control over their bodies and their lives?
What is spinal cord injury?
Your spinal cord is an information highway connecting your brain to the rest of your body (figure 1). Injuries to it are usually caused by sudden trauma, such as that sustained in sports or car accidents, and result in dislocation and / or breakage of vertebrae, which rip into the spinal cord tissue, damaging or severing axons. Sensation and motor control are lost below the level of the injury (figure 2).
Multiple cell types die at or near the site of the spinal cord injury, due tosecondary effects of the trauma, such as changes in blood supply, immune responses and an increase in free radicals and excitatory neurotransmitters (see box on the secondary effects of spinal cord injury).
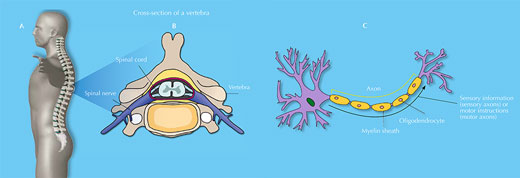
The spinal cord is a soft, jelly-like structure that extends from the base of the brain to the lower back (A). It is 38 to 43 cm long and, at its maximum width, is about as wide as a thumb. It sits in a hollow channel that runs through the spinal column’s 33 stacked vertebrae (B).
Spinal nerves (part of the peripheral nervous system) are connected to the cord at various intervals along its length and run to and from specific regions of the body. Together, the spinal cord and the brain make up the central nervous system.
The spinal cord consists of sensory and motor neurons (C). Sensory axons carry sensory information to the brain from the rest of the body, whereas motor axons carry motor instructions (both voluntary and involuntary) in the opposite direction. The cord’s neurons are supported by various glial cells, such as oligodendrocytes – which wrap myelin around axons, insulating them and enabling the quick and effective transmission of axon potentials – and astrocytes – which have multiple functions, such as providing nutrients and releasing growth factors for the benefit of other neural cells.
Images courtesy of Debivort / Wikimedia Commons (vertebra); Faigl.ladislav / Wikimedia Commons (neuron); Barnabé-Heider & Frisén (2008), with permission from Elsevier (grey man)
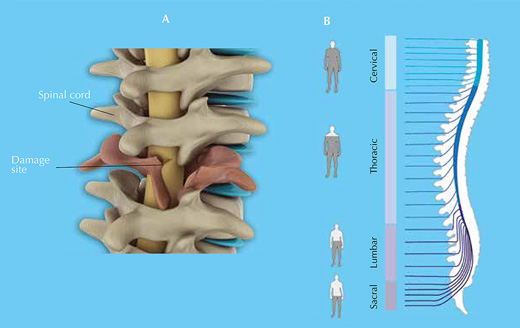
Click on image to enlarge.
Image courtesy of Barnabé-Heider & Frisén (2008), with permission from Elsevier
Secondary effects of spinal cord injury
Secondary effects that take place during spinal cord injury lead to death of multiple cell types, including neurons and oligodendrocytes. These secondary effects include:
- Ischemia: lack of proper blood supply (due to rupture of blood vessels). This reduces the supply of oxygen and nutrients to cells.
- Oedema: an accumulation of tissue fluid. This causes swelling and reduces the efficiency with which nutrients and molecules are exchanged between the blood and spinal cord cells.
- Immune invasion: injury triggers an inflammatory response that leads to an influx of immune cells (neutrophils, T-cells, macrophages and monocytes) to the spinal cord at and around the injury site. This response may be both protective (e.g. through the release of molecules that promote spinal cord repair, and the scavenging of damaged tissue) and detrimental (e.g. through inflammation that affects healthy tissue).
- Neurotransmitter release: damaged neurons release an excess of the excitatory neurotransmitter glutamate, which overexcites neural cells, killing them or stopping them from functioning.
- Free-radical release: cell damage and the inflammatory response increase the levels of free radicals, which further damage neural cells by reacting with molecules crucial for proper cellular function.
Existing treatments: damage limitation
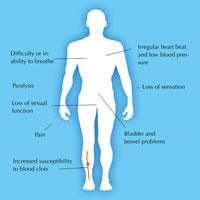
of spinal cord injury.
Click on image to enlarge.
Image courtesy of Nicola Graf
On its own, the body cannot replace the cells that are lost as a result of spinal cord injury, so its function is permanently impaired. Current short-term treatments consist merely of damage limitation: surgery to relieve the pressure on the spinal cord and to stabilise the spinal column, and immobilisation (by bracing) to prevent further damage. In the long term, patients are given physiotherapy and treatment to relieve injury-related symptoms, such as pain, as well as counselling to help them cope with their new disability (figure 3).
There is currently no cure for spinal cord injury, which causes such immense physical and emotional suffering. Several groups of scientists, however, are investigating the potential of stem cells to provide a cure.
Stem cell therapies
Stem cells are cells that can both differentiate into specialised cell types and self-renew to produce more stem cells. Broadly, there are two types: pluripotent stem cells, which can differentiate into any of the body’s cells, and adult stem cells, which can differentiate into only certain cell types. Pluripotent stem cells can be either extracted from embryos (embryonic stem cells) or generated in the lab (induced pluripotent stem cells) from specialised cells, such as skin cellsw2. Adult stem cells are found in various tissues, such as bone marrow.
There are two potential approaches to the use of stem cells in spinal cord injury:
- Transplantation of stem cells to the injury site.
- Recruitment of the injured spinal cord’s resident neural stem cells.
In both approaches, the intention is for stem cells either to replace lost or damaged spinal cord cells, or to promote recovery indirectly. This indirect benefit may come from the stem cells themselves, or from the cells into which they differentiate.
1) Transplanting stem cells
Studies of spinal cord injury performed on animal models (mainly rodents) have shown that transplantation therapy can help recovery, although it is often difficult to know which mechanisms are responsible. In these studies, three types of stem cell have been used: pluripotent stem cells, non-neural adult stem cells and neural stems cells (from either adults or embryos).
Pluripotent stem cells
Transplantation of pluripotent stem cells into animals has led to severe side effects, such as tumour formation. Before they are used to effectively treat spinal cord injury, therefore, these cells are treated to make them differentiate into neural progenitor cells – cells that have the potential to differentiate into particular types of specialised neural cells. One study showed that seven days after transplantation of oligodendrocyte progenitor cells into rats with spinal cord injury, axons were remyelinated and the rats were better able to move their legs (Keirstead et al., 2005).
Non-neural adult stem cells
Non-neural adult stem cells are extracted from various tissues, such as bone marrow, adipose tissue and the placenta. It is thought that these stem cells help repair the injured spinal cord indirectly. Many studies show that animals are able to better move and feel after transplantation of these cells (Parr et al., 2007).
Neural stems cells
Neural stem cells are extracted from certain parts of the nervous systems of embryos or adults. Many studies have shown that the transplanted cells differentiate into astrocytes that help new neural cells to grow (Enzmann et al., 2006; Pfeifer et al., 2006). Research published last year suggests that neural stem cells can re-programme the local inflammatory response to injury, reducing the proportion of harmful immune cells (such as macrophages), while promoting healing of the injured spinal cord (Cusimano et al., 2012). In most studies that have looked at recovery after neural stem cell transplantation, the animals were better able to move their legs.
2) Recruiting resident stem cells
Transplanting stem cells is risky: it requires a delicate surgical procedure, and (in the case of embryonic stem cells and neural stem cells) the immune system may reject the newly introduced cells. These risks could potentially be avoided using an alternative approach in which drugs instruct the injured spinal cord’s resident neural stem cells to promote recovery (Barnabé-Heider & Frisén, 2008).
Even if these risks can be overcome, research into this potential treatment, known as stem cell recruitment therapy, is still at an early stage and it remains to be seen whether it can aid recovery from spinal cord injury in animals.
Small changes can make a big difference
Understanding the mechanisms involved, testing the effectiveness in animals, running clinical trials in humans – the development of a treatment is a slow and complicated process. For now at least, transplantation therapy holds the greatest promise for the treatment of spinal cord injury with stem cells. In 2010, the Californian company Geron started a clinical trialw3 based on this approach, although it was halted at an early stage for financial reasons. Currently, Balgrist University Hospital in Zurich, Switzerland, is running a trialw4 using cells derived from human brain tissue. The hope is that when transplanted into the injured spinal cord, these cells may re-establish some of the circuitry important for the network of nerves that carry information around the body.
Given the multifaceted nature of spinal cord injury, it is unlikely that any one treatment will provide a cure, but even small improvements would make a big difference to patients’ lives. Imagine if, like Christopher Reeve, you were paralysed from the neck downwards: being able to move your arms and grip with your hands could make the difference between living a dependent or independent life.
Acknowledgements
This article was based on a fact sheet created for the Eurostemcell websitew5, and on the review paper Barnabé-Heider & Frisén (2008).
The author would like to thank Kate Doherty from Eurostemcell for her help in planning and writing the article. Thanks also go to Dr Stefano Pluchino of the Department of Clinical Neurosciences, University of Cambridge, UK, for his expert advice.
References
- Barnabé-Heider F, Frisén J (2008) Stem cells for spinal cord repair. Cell Stem Cell 3(1): 16-24. doi: 10.1016/j.stem.2008.06.011
- Cusimano M et al. (2012) Transplanted neural stem/precursor cells instruct phagocytes and reduce secondary tissue damage in the injured spinal cord. Brain. 135(2): 447-60. doi: 10.1093/brain/aws002
- Enzmann GU et al. (2006) Functional considerations of stem cell transplantation therapy for spinal cord repair. Journal of Neurotrauma 23: 479–495. doi: 10.1089/neu.2006.23.479
- Keirstead HS et al. (2005). Human embryonic stem cell-derived oligodendrocyte progenitor cell transplants remyelinate and restore locomotion after spinal cord injury. The Journal of Neuroscience 25: 4694–4705. doi: 10.1523/JNEUROSCI.0311-05.2005
- Parr AM et al. (2007). Bone marrow-derived mesenchymal stromal cells for the repair of central nervous system injury. Bone Marrow Transplant 40: 609–619. doi: 10.1038/sj.bmt.1705757
- Pfeifer K et al. (2006). Autologous adult rodent neural progenitor cell transplantation represents a feasible strategy to promote structural repair in the chronically injured spinal cord. Regenerative Medicine 1: 255–266. doi: 10.2217/17460751.1.2.255
Web References
- w1 – The Christopher and Dana Reeve Foundation is dedicated to curing spinal cord injury by funding research, and improving the quality of life of those with paralysis.
- The website includes videos of people with paralysis talking about their lives.
- w2 – In 2012, the Nobel Prize in Physiology or Medicine was awarded to John Gurdon and Shinya Yamanaka for their discovery that mature cells can be re-programmed to become pluripotent.
- Read more about embryonic and induced pluripotent stem cells on Nature Education’s Scitable website.
- In an online video on the Eurostemcell website, ‘Stem cells – the future: an introduction to iPS cells’, leading scientists tell the story of induced pluripotent stem cells.
- w3 – Read about the Geron clinical trial in the New York Times.
- w4 – Read more about the clinical trial at Balgrist University Hospital on the Clinical Trials website.
- w5 – Download the fact sheet Spinal cord injuries: how could stem cells help? from the Eurostemcell website.
- Eurostemcell’s stem cell toolkit is a set of downloadable, Creative Commons-licensed resources and activities suitable for a variety of educational settings. For example:
- The Embryonic stem cell research: an ethical dilemma fact sheet.
- Hope Beyond Hype is a story about stem cell therapies, from discovery to therapy, in the form of an interactive comic book.
- Ready or not? is a role play about spinal cord injuries, designed for classroom use.
Resources
- For a review of one of Eurostemcell’s DVDs for schools, see:
- Gebhardt P (2006) Review of A Stem Cell Story. Science in School 3: 88.
- Spinal cord injury: progress and promise in stem cell research is a short video featuring spinal cord injury patient advocate (and former footballer) Roman Reed.
- The website of the National Institute of Neurological Disorders and Stroke has detailed information on spinal cord injury.
- The International Campaign for Cures of Spinal Cord Injury Paralysis has statistics and other information on spinal cord injuries.
Review
This article focuses on the diverse consequences of spinal cord injury in humans, presenting cutting-edge science and considering potential future treatments based on stem cells.
Younger students (aged 11-14) could be motivated by the case of Superman actor Christopher Reeve to study the nervous system (e.g. the arc reflex). However, simplification of the text would be necessary.
The article would be particularly appropriate for upper secondary-school students (15-18 years old) due to the level of detail provided. It could be linked to topics including anatomy and physiology of the nervous system, physiology of the immune system, cell proliferation and cell differentiation. The list of suggested resources complements the article well.
The article could also be used as a starting point for interdisciplinary research projects linked to physics and chemistry. Topics for such projects could be: Why do injured cells release free radicals, and why are they harmful to cells? What molecules do free radicals react with primarily? Why does an oedema reduce the efficiency with which nutrients and molecules are exchanged between the blood and spinal cord cells? Which type of molecules might promote spinal cord repair?
The article could be used as the basis of comprehension and extension tasks including:
- Distinguish pluripotent stem cells from non-neural adult stem cells and neural stems cells (from either adults or embryos), considering their potential to generate specialised neural cells. Relate this to the mechanism of gene regulation.
- “Pluripotent stem cells can be either extracted from embryos (embryonic stem cells) or generated in the lab (induced pluripotent stem cells) from specialised cells, such as skin cells.” “Transplantation of pluripotent stem cells into animals has led to severe side effects, such as tumour formation”. Relate these sentences to the process of cell differentiation and genetic regulation.
- “Transplanting stem cells is risky: it requires a delicate surgical procedure, and (in the case of embryonic stem cells and neural stem cells) the immune system may reject the newly introduced cells.” Explain the biological mechanism that is responsible for this risk.
Betina da Silva Lopes, Portugal