Death Star or cancer tumour: proton torpedoes reach the target Understand article
A real-life version of proton torpedoes, popularized in Star Wars, offer an alternative to radiotherapy for the treatment of cancer.
In the first Star Wars movie, the Rebels are battling with the Empire’s Death Star, a giant space station that obliterates planets. It was heavily protected with ray shields and particle shields that deflected the laser blasts.
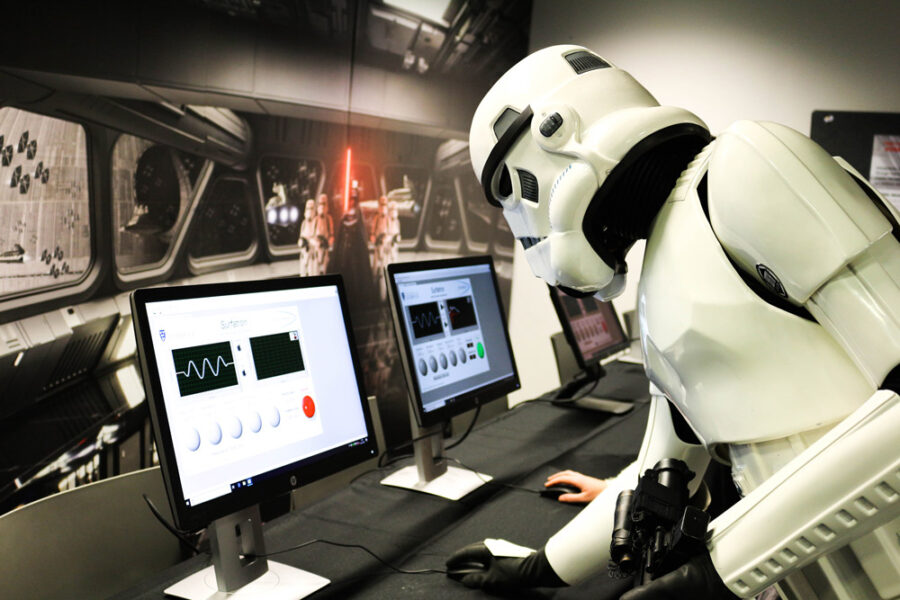
© Cockcroft Institute
Luke Skywalker fires ‘proton torpedoes’ through a gap into an exhaust port. The torpedo is directed towards its target to deliver its full energy deep within the Death Star, releasing a cloud of high-energy proton particles that initiate a damaging chain reaction. The mission is successful, and the Death Star is destroyed.
In real life, proton ‘torpedoes’ can be used to destroy a different enemy, cancer tumours, and offer many advantages over other forms of radiation therapy using X-rays.
Proton-beam therapy
Cancers grow through mutation of the host cell’s DNA. The cells replicate rapidly to form a tumour that is surrounded by healthy tissue. Radiation therapy using X-rays has been used for many years to destroy cancerous growths, but often at the expense of damage to healthy tissue, as most of the energy is deposited close to the skin.
In many cases, this is an acceptable risk, but in children this damage can result in secondary cancers as they get older, and for cancers near organs, careful targeting and control of the dose is critical to the success of treatment.
In comparison, protons pass through the skin with minimal impact and deliver the maximum amount of energy to their target. The proton beam, which is formed from charged particles, can be controlled to trace the outline of the tumour, ensuring that all affected tissue is destroyed.
Protons are also effective at damaging the cancerous DNA beyond repair. Protons are a form of directly ionizing radiation – they interact with cellular atoms or molecules and produce free radicals that create complex breaks to the double strands of DNA.
Medical accelerators
It was Sir William Henry Bragg who first observed that protons released a burst of energy as they reached the end of their path. This phenomenon is called the ‘Bragg peak’, and the depth of the peak can be precisely controlled by varying the amount of energy the proton beam is given. It is, therefore, a powerful way to target deep-seated, localized tumours.
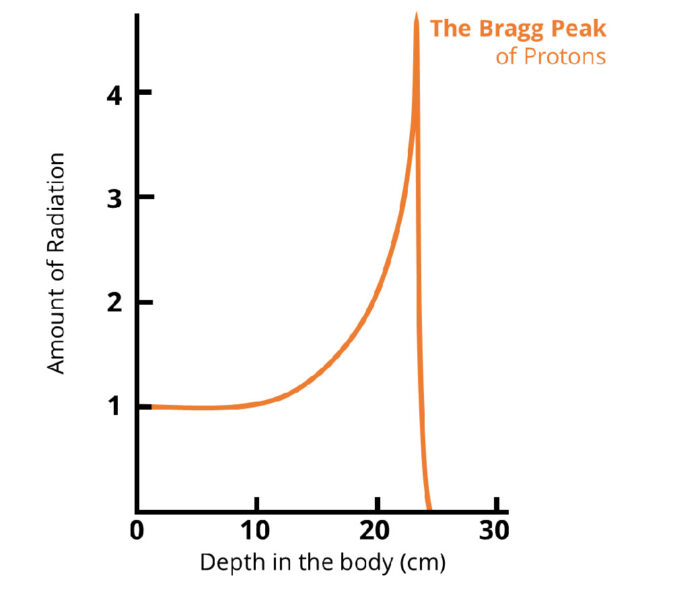
© Department of Physics, University of Liverpool
Bragg used natural ion sources and his protons could be stopped with a piece of paper. It quickly became clear that greater acceleration would be required to reach tumours located inside of a patient’s body.
This came with the invention of the cyclotron by Ernest Orlando Lawrence. It accelerates charged particles outwards from the centre of a vacuum chamber along a spiral path. The particles are held in a spiral trajectory by a static magnetic field and accelerated by a rapidly varying (radio frequency) electric field. When the beam of ions reaches the magnetic field boundary, they are extracted and guided into an external beam line. From there, the beam can be used for treatment purposes. Lawrence was awarded the Nobel Prize for his invention in 1939.
Cyclotrons were the most powerful accelerators for many years, but they had an energy limit of about 10 megaelectronvolts (MeV) for the acceleration of protons. A breakthrough was achieved simultaneously by two scientists working independently: Vladimir Veksler in Moscow and Edwin McMillan in the USA. They shared the Nobel prize for showing that, by adjusting the frequency of the accelerating voltage to the decreasing frequency of rotating protons, it was possible to accelerate protons to several hundred MeV. Their discovery, the ‘synchrotron principle’, proved that there was no theoretical limit to the energy to which particles could be accelerated.
With a circumference of 27 km, the largest synchrotron in operation is the Large Hadron Collider (LHC), which uses a linear accelerator to shoot a beam of protons into a series of circular accelerators that boost the speed of the protons until they reach maximum energy in the LHC.
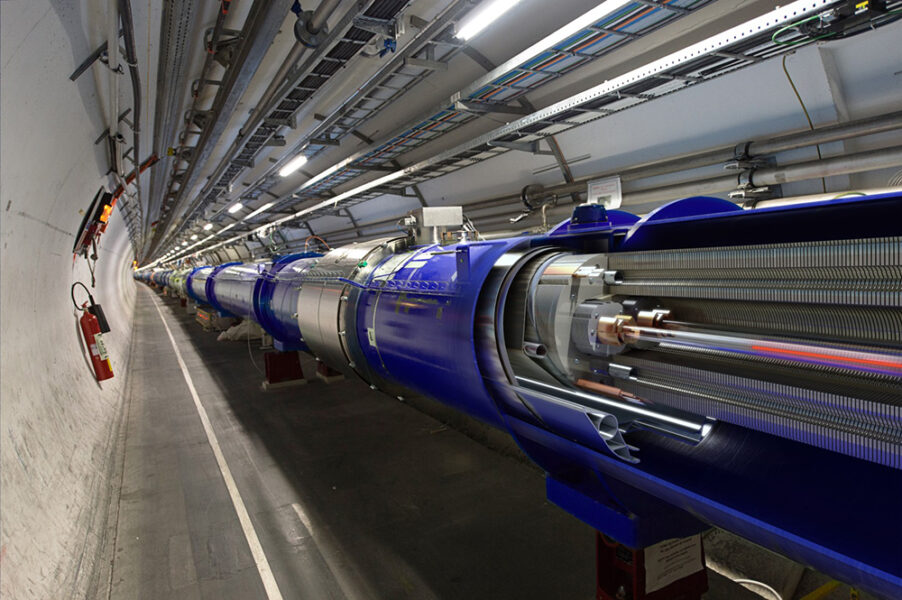
© Daniel Dominguez/CERN
The application of discovery science to a real-life setting often needs the perspective of outsiders. The ‘father of proton therapy’ is Robert Wilson, who published a paper titled “Radiological use of fast protons” in a medical journal in 1946.[1] Wilson realized that, by utilising the Bragg-peak phenomenon, a proton beam could be controlled to penetrate deep within the body and deposit its maximum energy into the tumour, sparing healthy tissue.
The place where the protons stop inside the body can be determined by knowing the energy at which they are fired from the particle accelerator. This allows fine control over where most of the radiation dose is delivered. For a higher dose inside the body, a heavier ion, such as helium or carbon, can be used, which will be able to deposit more energy.
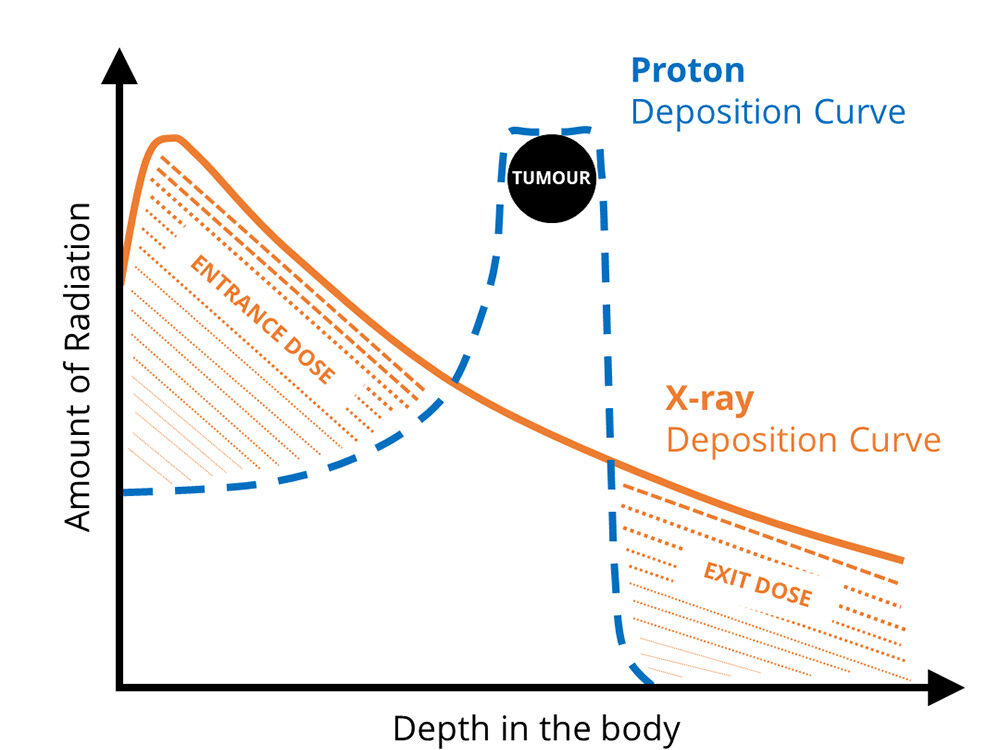
© Department of Physics, University of Liverpool
This insight has been developed further in recent years. With a magnetic scanning system, the beam spot can be controlled very precisely to sculpt the dose to the tumour.
Current research
High-energy proton-beam therapy is now becoming available in the UK, following the opening of a therapy unit at the Christie Hospital in Manchester in 2018, and further facilities are planned, for example, in London, Liverpool, and Oxford. Internationally, there are almost 100 proton-therapy centres in operation and over 200 000 patients have been treated worldwide. However, these facilities are costly. The high-energy proton beams require a significant investment in particle accelerator and building infrastructure.
To overcome this issue, the Optimization of Medical Accelerators (OMA) project is looking at ways to improve control of the beams, optimize the treatment process, and reduce the size of the accelerators required. For example, accelerator scientists and engineers at the Cockcroft Institute have been working with clinical teams at the Clatterbridge Cancer Centre on beam-transport optimization studies, diagnostics developments, and Monte Carlo simulations into dose-delivery scenarios.
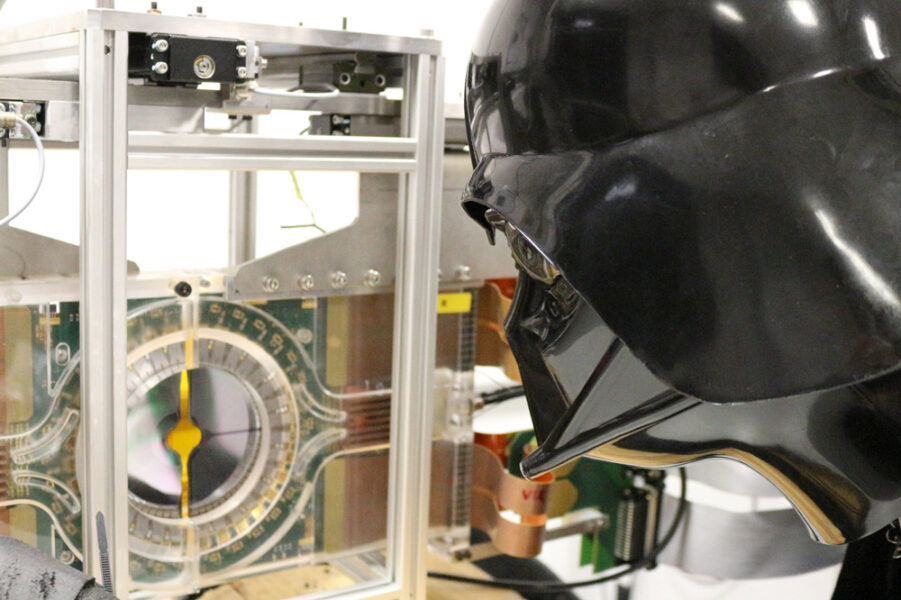
© Cockcroft Institute
Monte Carlo simulations are used to estimate the possible outcomes of an uncertain event and can be used to predict the average behaviour (i.e., energy-deposition pattern) of a proton beam that is guided by electromagnetic fields. Monte Carlo dose calculations are found to be more accurate than analytical algorithms because of the more detailed consideration of tissue interaction.
Beam optimization uses instruments originally developed for accelerators, such as the LHC, to measure the detailed properties of the treatment beam without touching it. This helps to target tumours with more precision and helps to reduce machine setup times, allowing the treatment of more patients.
By working in multidisciplinary teams, it is possible to combine therapies to provide a more personalized approach to cancer care; this can include surgery, conventional radiotherapy, chemotherapy, gene therapies, and methods like proton or ion therapy.
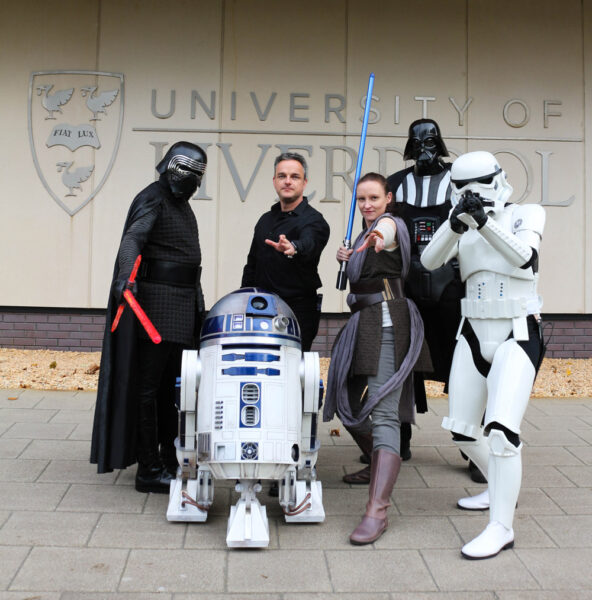
© Cockcroft Institute
Medical physicists are an important part of this team, and new imaging techniques and maybe even portable accelerators will add to the armoury in the fight against cancer. May the Force be with them!
References
[1] Wilson R (1946) Radiological use of fast protons. Radiology 47:487–491 doi: 10.1148/47.5.487.
Resources
- Discover how particle therapy works; the video explains different elements that make a cutting-edge clinical centre.
- Learn more about the medical applications of accelerator science.
- Watch a video lecture on Proton Beam Therapy: How the Large Hadron Collider Cures Cancer. An extended video provides context on how the LHC can be used to cure cancer and explains research within the OMA network.
- Learn how Star Wars can be used to engage students with accelerator science: Welsch C P (2021) The physics of Star Wars: introducing accelerator science. Science in School 54.