Fusion in the Universe: when a giant star dies… Understand article
Péter Székely from the University of Szeged, Hungary, and Örs Benedekfi from the European Fusion Development Agreement in Garching, Germany, investigate how a star dies and what a nearby supernova explosion would mean for us on Earth.
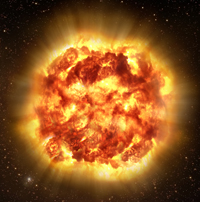
supernova explosion
Image courtesy of ESO
At 7:35 in the morning of 23 February 1987, a kilometre below the ground, the Japanese neutrino detector Kamiokande II registered 11 neutrinos in 15 seconds. This does not sound very dramatic, but neutrinos are hard to detect because they interact very weakly with matter. Normally, the detector registers only a couple of neutrinos per day from the Sun, so this indicated a spectacular event somewhere in the Universe: a giant star had died.
Size is everything
Probably the single most important property determining the fate of a star is its mass. As described earlier in this series (Boffin & Pierce-Price, 2007), stars with a similar mass to our own Sun die without any upheaval: they fuse helium into carbon and oxygen, then release their outer layers as a planetary nebula and over billions of years, the core cools down to form a white dwarf.
More massive stars have a shorter lifetime and more violent destiny. Whereas a star the size of our Sun can live for billions of years, stars that are eight to ten times the mass of our Sun last only millions of years because they rapidly run out of fuel. When this happens, the equilibrium is lost between two fundamental forces: gravity, which tends to contract the matter of the stars; and radiation pressure produced by nuclear fusion reactions in the core, which tends to expand the star. The core contracts to form a neutron star and the outer layers of the star fall inwards and rebound from the very dense core in a gigantic explosion: a Type II supernova.
Waves of particles, including neutrinos, leave the core, carrying the gravitational energy of the collapsing star. The infalling outer layers of the star absorb many of these neutrinos, giving rise to extremely high temperatures – hot enough to trigger the fusion of elements including gold and uranium (as described in Rebusco et al., 2007). A small proportion of these neutrinos, however, escapes the atmosphere of the dying star and can be detected on Earth, in the silence deep below the planet’s surface.
A second type of stellar cataclysm occurs during the collision of a white dwarf and a much larger red giant with an expanded atmosphere (hundreds of millions of kilometres in diameter). If a white dwarf and a red giant orbit each other in a close relationship known as a binary star, the white dwarf can accrete material from its companion and increases in mass (see image). Once the white dwarf exceeds 1.44 times the mass of our Sun (the Chandrasekhar limit), it becomes unstable, fusion reactions produce heavy elements such as lanthanum and ruthenium, and the whole disintegrates in a giant explosion: a Type I supernova (see image).
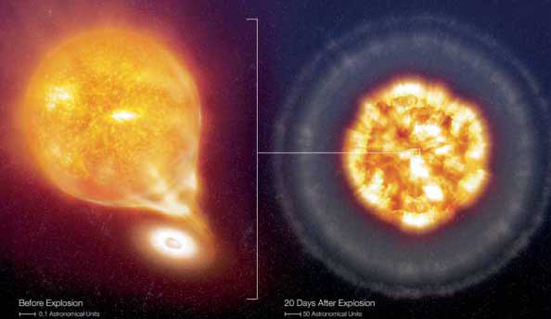
Once the mass of the white dwarf has reached a critical limit, the star explodes as a Type Ia supernova
Image courtesy of ESO
Whether Type I or II, a supernova is one of the most powerful events in the Universe since the Big Bang. In the weeks after the explosion, the star and its remnants emit more energy than our Sun does over billions of years. This enormous explosion can outshine all other stars in its host galaxy and the light can be detected from thousands of millions of light years away. Indeed, a supernova occurring anywhere in the Universe will probably be visible with a powerful telescope.
The remains of a star
So what remains after this violent and dramatic event? In the centre of the explosion of a star that is less than 20 solar masses is a newly formed neutron star, with the mass of the star packed into a sphere with a radius of only ten kilometres – unimaginably dense. The weight of one teaspoon of neutron star equals that of all the traffic vehicles (millions of cars, buses, trains) we have here on Earth (Swinton, 2006). Due to conservation of angular momentum (the smaller the radius, the faster the rotation), neutron stars often spin very rapidly (hundreds of revolutions per second); this may cause beams of radio waves emanating from the magnetic poles, in which case we describe the star as a pulsar.
An even more exotic kind of remnant than a neutron star or pulsar is a black hole, born when a star of at least 20 solar masses explodes in a Type II supernova. When such a massive star dies, the gravitational collapse does not stop with the formation of a neutron star – instead, the infalling matter produces a very curious phenomenon with a theoretical volume of zero and infinite density. Nothing can leave the black hole or its close surroundings unless it exceeds the speed of light, but no light can escape the immense gravitational pull – hence the name.
As well as a neutron star or black hole, we can often observe the remnants of the star’s gas clouds, which were blasted away by the supernova. One of these interesting objects is the Crab nebula: the remnant of the supernova that occurred around 5500 BC and was observed in 1054 by Chinese astronomers. Like the other remnants, it will disperse into the interstellar space over thousands of years.
Learning from supernovae
Even without the benefit of modern telescopes, a supernova in a nearby galaxy is hard to miss. The earliest observed supernova was recorded by Chinese astronomers in 185 AD. When the formation of the Crab nebula was detected in China some 800 years later, this ‘guest star’ was so bright that it could be seen in broad daylight for weeks.
The maximum brightness of the individual supernovae explosions is very similar (we call them ‘standard candles’) because the exploding masses are similar. By comparing the expected brightness with the observable brightness, we can calculate how far away the host galaxy of the supernova is. This technique is very important in the ‘cosmic distance ladder’: it is so far the best method for measuring the distance of distant galaxies. And of course, it is one way to observe the early history of the Universe: by the time we see a distant cataclysm, the star itself has long since died.
Of course, astronomers not only want to know how far away a supernova’s host galaxy is, but also want to characterise the individual supernova itself. They therefore use two indirect methods: photometry and spectroscopy. Photometry measures the diminishing brightness of the supernova over time and the maximum brightness: this is the technique used to calculate the distance of faraway galaxies. Spectroscopy allows the chemical elements in the supernova to be deduced on the basis of their characteristic wavelengths (as explained in Westra, 2007). Usually astronomers apply both methods to determine the physical properties of the dying star, such as its mass, temperature and luminosity.
Nearby supernovae?
So far, all observed supernovae have been a long way from Earth, but what would happen if a closer star became a supernova? Fortunately, supernovae are fairly rare, with only one supernova every 50 to 100 years in an ordinary spiral galaxy like the Milky Way. The last supernovae observed in the Milky Way were in 1572 and 1604, although vast and dense dust clouds could have hidden some explosions on the far side of our galaxy. The most recent and relatively close cataclysm was SN 1987A, which in 1987 ignited in the Large Magellanic Cloud, one of the smaller escort galaxies of the Milky Way, at a distance of around 160 000 light years (see image); this explosion was visible to the naked eye. Further afield, astronomers observe hundreds of supernovae explosions per year in other distant galaxies, sometimes two at the same time in the same galaxyw1.
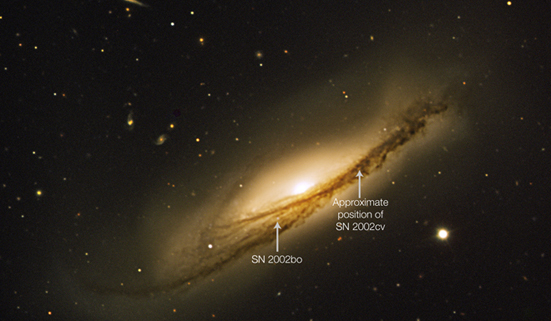
Image courtesy of ESO
Fortunately, there is no star in our immediate neighbourhood (up to about 12 light years) that will turn into a supernova in the foreseeable future, although further away, astronomers have already identified some potential supernovae. Betelgeuse, the red supergiant at the left shoulder of the constellation Orion, is the best candidate, but this is around 450 light years from us. We think the safety zone is around 100 light years across: closer than this, and a supernova could seriously damage our planet. Of more concern is IK Pegasi – a binary star consisting of a white dwarf and an ageing normal star about 150 light years from us. Whereas Betelgeuse could blow up at any time from tomorrow to thousands of years hence, IK Pegasi will meet its fate sometime in the next few million years.
Effects on Earth
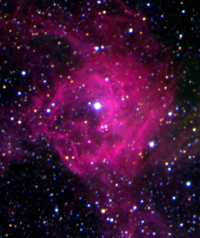
(SNR 0543-689) is the
remnant of a recent
supernova explosion
Image courtesy of ESO
So what effects would a nearby supernova have on Earth? Supernovae produce vast amounts of gamma radiation and particles such as protons and electrons, all of which have very high energy and could destroy the Earth’s atmosphere by degrading ozone and atomic oxygen. For example, gamma rays dissociate atmospheric nitrogen (N2) into nitric oxide (NO) and other nitrogen oxides (NOx) that catalyse the breakdown of ozone.
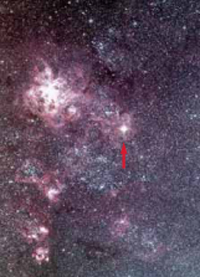
Magellanic Cloud (marked
with an arrow)
Image courtesy of ESO
Without the protective ozone layer, ultraviolet rays from our own Sun would reach the Earth’s surface unobstructed and destroy phytoplankton (microscopic plants that live in the water column). As phytoplankton form a fundamental component of the food chain, their loss would have a devastating effect on most other organisms. High-energy radiation would also damage living cells, causing cancer and genetic mutations: rather like a very heavy dose of X-rays. It is also possible that levels of radioactive elements in the atmosphere could increase, with harmful effects.
It may have been a supernova blast that caused the Ordovician-Silurian mass extinction approximately 450 million years ago. More than half of marine life forms were eradicated in this event, believed to be the second largest extinction event on Earth in terms of the number of genera that died out. It is believed that the extinction was a result of both the destruction of phytoplankton and the reduction in global temperatures caused by the opacity of nitrogen dioxide (NO2).
It is also possible that Earth suffered a close supernova explosion about 2.8 million years ago. During its explosion, a dying star unleashes a wave of radioactive elements which can be deposited on the surface of planets. Characteristic radioactive elements, for example iron-60, have been found when deep cores were drilled from the sea floor. These may be evidence of a supernova, but the debate is ongoing. More evidence of a recent and local supernova explosion is the Local Bubble, a cavity 300 light-years wide in the interstellar medium where our own Solar System is located. This bubble was created by various supernovae explosions, which pushed away the thin surrounding interstellar medium.
Our supernova origins
Fortunately those giant explosions have positive effects as well. We probably owe our existence to a nearby supernova. The shock wave from a supernova compresses the surrounding interstellar matter – a vast but thin cloud of dust, atomic and molecular gas – and triggers star formation. So perhaps a supernova caused the collapse of the enormous cloud from which the Solar System was born.
Furthermore, supernovae were the origin of everything we know: humans and everything from the smallest bacterium to the highest mountain are made from the ashes of stars. A normal star can produce lighter elements, but the fusion reactions forming heavier elements require the enormous temperatures and pressures prevailing in giant stars (for more details, see Boffin & Pierce-Price, 2007; Rebusco et al., 2007). These elements are formed and dispersed into interstellar space by supernovae, enriching the clouds of matter from which stars, planets and life arise. In a sense, we are children of supernovae.
Supernovae, therefore, have shaped our Universe and our history. They created the conditions for life on Earth by producing elements, they affected evolution by causing mass extinctions and now, by examining supernovae, we can learn a lot about the Universe and ourselves.
EFDA education and outreach
Many of the fusion research institutes in the European Fusion Development Agreement (EFDA) have their own outreach programmes, which often include lectures, and visits to schools and research facilities such as JET. Details of the individual research institutes are available on the EFDA websitew2. Within the framework of EIROforumw3, EFDA participates in Science in School, the Science on Stagew4 festival and other outreach and education projects.
EFDA has produced a 60-page brochure for secondary schools, ‘Energy, Powering Your World’, giving a broad introduction to the world of energy. Topics include the ways we use energy in our daily lives, where it comes from, and how we will deal with our energy needs in the future.
To receive a free printed copy of the brochure, available in English, Dutch, Spanish, French, German or Italian, send an email to aline.duermaier@efda.org, including your name, postal address and the number of copies you would like (up to five). The brochure can also be downloaded from the EFDA website.
EFDA brochure for secondary schools
EFDA has a range of other educational materials available, such as a CD-ROM, ‘Fusion, an energy option for the future’, and a general poster on fusion, both of which can be requested via the EFDA website. The website also provides basic and more advanced information about fusion science.
References
- Boffin H, Pierce-Price D (2007) Fusion in the Universe: we are all stardust. Science in School 4: 61-63.
- Rebusco P, Boffin H & Pierce-Price D (2007) Fusion in the Universe: where your jewellery comes from. Science in School 5: 52-56.
- Swinton J (2007) The neutron teaspoon. Science in School 3: 92.
- Westra MT (2007) A fresh look at light: build your own spectrometer. Science in School 4: 30-34.
Web References
- w1 – To discover when and where the latest supernovae have detonated, see the Supernovae website, where scientists and amateurs hunt and register new supernova explosions.
- w2 – The European Fusion Development Agreement (EFDA) website
- w3 – EIROforum website
- w4 – Science on Stage website