A hole in the sky Understand article
Twenty-five years ago, the discovery of the hole in the ozone layer hit the news. How have things developed since? Tim Harrison and Dudley Shallcross investigate.
Discovering the hole
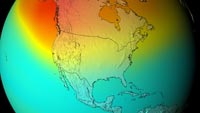
Goddard Space Flight Center
Scientific Visualization Studio
It was a serendipitous find, as Jonathan Shanklin, one of the hole’s discoverers, remembers: having joined the British Antarctic Surveyw1 in 1977, he was supposed to digitise their backlog of ozone measurements – until then, handwritten data sheets. As it turned out, this included the crucial decade, the 1970s, when ozone levels began to drop.
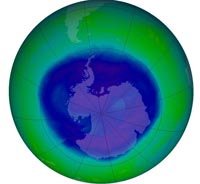
its annual maximum on 12
September 2008, stretching
over 27 million square
kilometres. This is
considered a moderately
large ozone hole, according
to NASA
Image courtesy of NASA
There had already been growing concern that industrial chlorofluorocarbons (CFCs) – organic compounds such as trichlorofluoromethane (CFCl3) and dichlorodifluoromethane (CF2Cl2), then widely used as refrigerants, propellants (in spray cans) and solvents – might destroy the ozone layer. For an open day in 1983, Shanklin prepared a graph – ironically to show that the ozone data from that year were no different from 20 years before. Although this was true for the overall ozone levels, he noticed that the springtime values did look lower from one year to the next. Further studies corroborated this, and in 1985 Shanklin and his colleagues Joe Farman and Brian Gardiner published their findings: each Southern Hemisphere spring, a hole gaped in the ozone layer above the Antarctic, it was probably caused by CFCs, and it was growing (Farman et al., 1985).
What is the chemistry behind this, and why is the ozone hole dangerous?
Ozone in the stratosphere
Ozone (O3) is a much less stable triatomic form of oxygen (O2). It is a pale blue gas present at low concentrations throughout the atmosphere – and a double-edged sword: in the troposphere (see image below), ozone is an air pollutant which can damage the respiratory systems of humans and other animals and burn sensitive plants. The ozone layer in the stratosphere, however, is beneficial, preventing most of the harmful ultraviolet (UV) light emitted by the Sun from reaching Earth’s surface.
The rate of ozone formation maximises in the stratosphere, the second highest layer of Earth’s atmosphere (at about 10-50 km altitude; see image), through a photochemical mechanism:
O2 + hν → O• + O• λ ~ 200 nm (1)
O• + O2 + M → O3 + M (2)
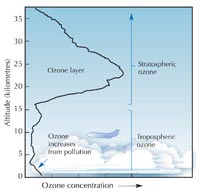
the lower atmosphere. Most
ozone is in the stratospheric
ozone layer. Near Earth’s
surface, the ozone levels
increase as a result of
pollution from human
activities. Click to enlarge
image
Public domain image; image
source: Wikimedia Commons
An oxygen molecule (O2) absorbs a photon of UV light (hν) with a wavelength (l) around 200 nm and dissociates into two oxygen atoms (O•) (reaction 1). Each of these can then combine with another oxygen molecule to form ozone, if the pressure (M) is high enough (approximately one thousandth of an atmosphere) to stabilise the newly formed ozone molecule (reaction 2). The higher the altitude, the faster the rate of reaction 1 (below 20 km altitude, no 200 nm photons occur because they have all been absorbed in reaction 1). The rate of reaction 2, however, is faster closer to the ground, where atmospheric pressure is higher. As a result, the maximum amount of ozone is created between about 25 and 30 km altitude (see graph).
The stratosphere has two important consequences for life on Earth. First, ozone itself absorbs high-energy UV radiation at around 250 nm (reaction 3):
O3 + hν → O• + O2 λ ~ 250 nm ΔH = – 90 kJ mol-1 (3)
Between them, oxygen (reaction 1) and ozone (reaction 3) therefore filter out of the atmosphere most of the short-wave UV radiation between 200 and 300 nm, which would otherwise be very damaging to life on Earth.
Second, reaction 3 produces a lot of heat, so the stratosphere is a warmer layer than the top of the troposphere (see image below), making the weather in the troposphere less extreme than it would otherwise be.
Reactions 2 and 3 rapidly interconvert oxygen atoms and ozone. There is another slow reaction, though, which is known to destroy both oxygen atoms and ozone, namely the reaction between these two species:
O• + O3 → O2 + O2 (4)
Reactions 1-4 are summarised in the diagram below.
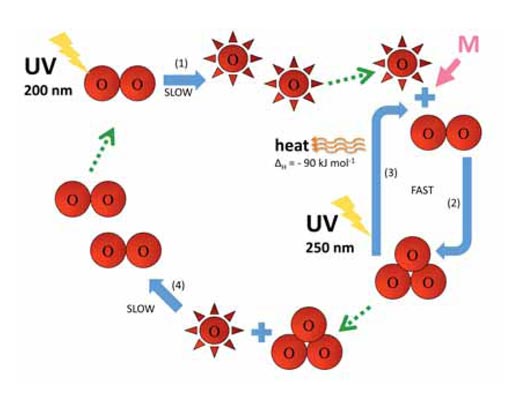
Image courtesy of Marlene Rau
Natural catalytic cycles reduce the levels of ozone
In 1995, Paul Crutzen, Mario Molina and F Sherwood Rowland were awarded the Nobel Prize in Chemistry for their work on the formation and decomposition of ozone in the stratosphere. What had they learned? In the 1970s, Crutzen and others discovered the existence of natural catalytic cycles that speed up reaction 4 and reduce the amount of ozone in the stratosphere (Crutzen, 1970, 1971): water (H2O), methane (CH4), nitrous oxide (N2O) and chloromethane (CH3Cl) are released into the atmosphere from biological processes occurring on Earth’s surface, and lead to the formation of radicals such as hydroxyl (OH•), nitric oxide (NO•) and chlorine (Cl•), which catalyse the decomposition of ozone.
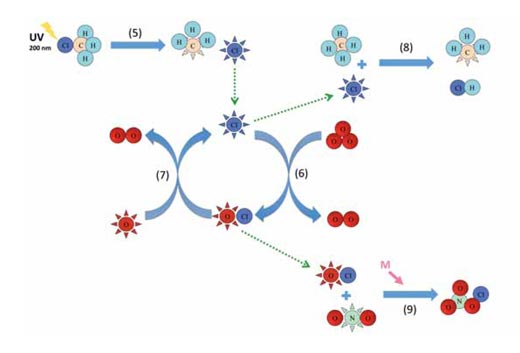
Image courtesy of Marlene Rau
Reaction 5 shows how chloromethane releases chlorine radicals into the stratosphere through photolysis, and reactions 6 and 7 are an example of a catalytic cycle (see diagram above). The reactions of the other catalysts are analogous with reactions 6 and 7. Chloromethane is released in part by both marine and terrestrial organisms, such as red macroalgae, white rot fungi and higher plants, to regulate chloride ion levels in the cells and – after 30 to 40 years – can reach the upper stratosphere (around 40 km altitude) where it is broken down by sunlight (photolysis):
CH3Cl + hν → •CH3 + Cl• l ~ 200 nm (5)
The resulting chlorine free radical (Cl•) can then participate in a catalytic cycle:
Cl• + O3 → ClO• + O2 (6)
ClO• + O• → Cl• + O2 (7)
Reactions 6 and 7 taken together are in fact equivalent to reaction 4, but happen much faster – in the case of the chlorine / chlorine monoxide (ClO•) radical cycle, about 30 000 times faster. So why do these catalytic cycles not destroy all the ozone? The answer lies in the termination of these cycles via the formation of stable molecules:
Cl• + CH4 → •CH3 + HCl (8)
ClO• + •NO2 + M → ClONO2 + M (9)
Eventually, a chlorine free radical will encounter a methane molecule and react to form hydrochloric acid (HCl, reaction 8). Similarly, a chlorine monoxide radical will bind to a nitrogen dioxide radical, forming chlorine nitrate (ClONO2, reaction 9) – another pressure-dependent reaction that therefore works better at lower altitudes. Both hydrochloric acid and chlorine nitrate are very stable, and the removal of chlorine and chlorine monoxide radicals eventually stops the catalytic cycle.
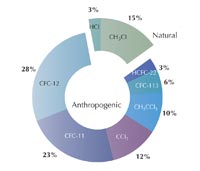
chlorine according to WMO /
UNEP Scientific Assessment
of Ozone Depletion: 1998.
Click to enlarge image
Image courtesy of Andrew
Ryzhkov; image source:
Wikimedia Commons
The Antarctic ozone hole puzzle
It was not long before scientists realised that CFCs could trigger a similar catalytic cycle of ozone degradation: in 1974, Molina and Rowland not only warned that levels of CFCs continued to increase without regulation, but also predicted that CFCs would cause a significant additional loss of ozone at around 40 km altitude (see Molina & Rowland, 1974). However, when the ozone hole was finally found in 1985, it was in fact at around 20 km altitude, over the South Pole in the Southern Hemisphere springtime (see Farman et al., 1985).
It soon emerged that chlorine free radicals from the CFCs were responsible, but many questions remained unanswered. Why did the hole occur over the Pole? If it occurred over the South Pole, why not also over the North Pole? Why only in spring? And why was the ozone hole at 20 km altitude instead of at 40 km, as predicted? After all, CFCs could not be broken down by sunlight at an altitude as low as 20 km, since the photon density was insufficient. For the same reason, not enough oxygen atoms are produced at this altitude for reaction 7 to occur. Many years of further research revealed the complete story.
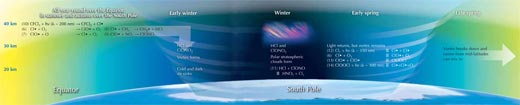
Image courtesy of Dudley Shallcross, Tim Harrison, Marlene Rau and Nicola Graf
First, chlorine free radicals released from the CFCs, e.g.
CFCl3 + hν → •CFCl2 + Cl• l ~ 200 nm (10)
could react with methane (reaction 8) forming hydrochloric acid, or with ozone (reaction 6) forming chlorine monoxide radicals, and through reaction 9 could subsequently form chlorine nitrate. This sequence of reactions would increase the concentrations of hydrocholoric acid and chlorine nitrate at around 40 km altitude globally.

leading to space
Public domain image; image
source: Wikimedia Commons
Each Southern Hemisphere winter, the South Pole is plunged into darkness for approximately three months. The air in the stratosphere above the South Pole cools down; without UV radiation, reaction 3 does not occur, so no heat is released. The air sinks and Earth’s rotation causes it to spin and form a vortex as it does so, like water going down a plughole. This vortex is so strong that no air from outside can get in, and no air from inside can get out. Air that is rich in hydrochloric acid and chlorine nitrate from 40 km altitude is drawn down into this cold and dark vortex.
In the extreme cold of the polar winter, the air in this vortex becomes so cold that below -78°C (195 K) and at an altitude of 15-25 km, polar stratospheric clouds form from water and/or acid ice crystals.
The first peculiar bit of chemistry is that hydrochloric acid and chlorine nitrate can adsorb onto polar stratospheric clouds and undergo a fast heterogeneous reaction from gaseous to solid phase, producing nitric acid (HNO3) that becomes incorporated into the ice crystals, whilst the chlorine (Cl2) is released back into the gas phase.
HCl + ClONO2 → HNO3 + Cl2 polar stratospheric clouds (11)
This reaction can take place all winter, if it is cold enough to form polar stratospheric clouds. When the sunshine returns in spring, there are plenty of chlorine molecules at around 15-25 km altitude, which are photolysed to produce chlorine radicals:
Cl2 + hν → Cl• + Cl• l ~ 350 nm (12)
and subsequently chlorine monoxide radicals via reaction 6.
However, in the polar spring, reaction 7 (the formation of chlorine radicals and oxygen molecules from chlorine monoxide radicals and oxygen radicals) is very slow, since there are so few oxygen atoms present due to the lack of 200 nm photons at this altitude, and here is where a second peculiar piece of chemistry occurs. At low temperatures, such as in the polar vortex – which is still very cold even in spring – chlorine monoxide radicals can form a dimer, chlorine peroxide (ClOOCl):
ClO• + ClO• → ClOOCl (13)
This dimer is unstable at room temperature but forms quite readily at low temperatures (below -30 °C) and can be photolysed:
ClOOCl + hν → Cl• + O2 + Cl• l ~ 300 nm (14)
So even though reaction 7 cannot occur, reaction 14 provides a way to regenerate chlorine free radicals with the help of light, and the catalytic cycle for ozone depletion can start in earnest now that the sunshine has returned.
In what way does this differ from the natural catalytic cycles we looked at before – why is there a total removal of ozone at some altitudes in this vortex? First, reaction 8 (which removes chlorine radicals and can terminate the cycle) is very slow at the low temperatures found in the vortex, and therefore ineffective. Second, all the nitrogen dioxide required for reaction 9 (which could likewise terminate the cycle, through the formation of ClONO2) has been converted to nitric acid throughout the winter (e.g. through reactions 9 and 11) and it is not available to be regenerated since there is no upward flow in the vortex (at the base of the vortex, air flows from the South Pole to the Equator, where the upward flow takes place). Therefore the cycle carries on unchecked and destroys all the ozone at that level. Finally, without ozone, reaction 3, which would otherwise warm this region, is absent, and so the vortex lasts well into the spring, exacerbating the ozone depletion.
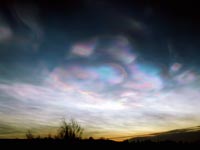
Asker, Norway
Public domain image; image
source: Wikimedia Commons
The only reason that the ozone hole is more severe over the South Pole than the North Pole is that the spring temperatures in the stratosphere above the North Pole are slightly warmer than those above the South Pole, because there are more mountain ranges in the mid to high latitudes of the Northern Hemisphere, which change the dynamics of atmospheric flow, so there are fewer polar stratospheric clouds.
In late spring, the flow of ozone-rich air from above eventually warms the vortex via reaction 3, allowing the vortex to eventually break down. Since exchange with other parts of the atmosphere then becomes possible again, the ozone hole is filled with ozone from the surrounding air.
In some years, the ozone hole over Antarctica has grown large enough to reach Australia, New Zealand, Chile and Argentina, growing to 1.5 times the size of the USA; and when the ozone hole breaks up, the ozone-depleted air drifts out into nearby (populated) areas, including South Africa. For the people in these countries, the ozone hole poses a direct health threat. The main concern is the increased exposure to UV, which may cause skin cancer and ocular cortical cataracts, as well as damage to the immune system. Furthermore, excessive UV radiation damages plants and building materials.
CFCs and ozone today
Today, we have a good understanding of the physics and chemistry governing the ozone layer.
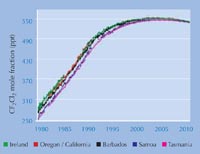
concentration of CFCs
increased rapidly and is now
slowly decreasing again. Data
are given for CF2Cl2 , at the
five AGAGE surface
measurement stations from
1978 to the present day,
taken from the AGAGE
websitew2 in September
2010. Click to enlarge image
Image courtesy of the AGAGE
project
Once the true impact of CFCs on ozone depletion became apparent, governments passed regulations to stop the use of CFCs, replacing them with alternative, shorter-lived, species (hydrofluorocarbons and hydrochlorofluorocarbons), which were to be phased out eventually too: the Montreal Protocol of 1987 and especially its amendments in 1990 and 1992, which speeded up the phase-out, were an environmental success.
The most recent data from AGAGE (the Advanced Global Atmospheric Gases Experiment)w2, which has been monitoring levels of CFCs and their replacements since 1978, shows that even the atmospheric levels of dichlorodifluoromethane (CF2Cl2), the longest-lived CFC, are now decreasing: the legislation has been effective (left). An ozone hole still forms each spring over the South Pole, but estimates are that by 2050 this will no longer happen, and that by 2080 the global ozone will return to 1950s levels.
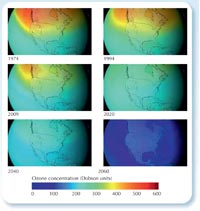
would look if CFCs had not
been banned. Click to
enlarge image
NASA images courtesy of the
Goddard Space Flight Center
Scientific Visualization Studio
The ozone hole is the result of an increased use of CFCs, which began in the 1930s – like any other gas, CFCs take 30-40 years to reach the upper stratosphere, which means that there is a corresponding lag in their effect on the ozone layer. We are currently experiencing the stratospheric chlorine peak resulting from the highest levels of CFC use in the 1980s – so the maximum size the ozone hole reaches each year should begin to decrease a few years from now.
Although recovery is slow, we have definitely stopped a disaster: scientists have calculated that if the use of CFCs had continued at its 1970s growth rate of 3% per year, this would have led to a global ozone hole by 2060, with all the health problems that would bring (see right; Newman et al., 2009).
Perhaps the most important lesson to be learned from the ozone hole is just how quickly our planet can change as a result of human impact – especially for the worse, but also for the better – and that change is possible if we take action concertedly, effectively and quickly.
References
- Crutzen PJ (1970) Influence of nitrogen oxides on atmospheric ozone content.
- Quarterly Journal of the Royal Meteorological Society 96: 320-325. doi: 10.1002/qj.49709640815
- Crutzen PJ (1971) Ozone production rates in an oxygen-hydrogen-nitrogen oxide atmosphere. Journal of Geophysical Research 76(30): 7311-7327. doi: 10.1029/JC076i030p07311
- Farman JC, Gardner BG, Shanklin JD (1985) Large losses of total ozone in Antarctica reveal seasonal ClOx/NOx interaction. Nature 315: 207-210. doi: 10.1038/315207a0
- The article is freely accessible on the Nature website (www.nature.com) or via the direct link: http://tinyurl.com/2wemxhn
- Molina MJ, Rowland FS (1974) Stratospheric sink for chlorofluoromethanes – chlorine atomic-catalysed destruction of ozone. Nature 249: 810-812. doi: 10.1038/249810a0
- The article is freely accessible on the Nature website (www.nature.com) or using the direct link: http://tinyurl.com/2u69cul
- Newman PA et al. (2009) What would have happened to the ozone layer if chlorofluorocarbons (CFCs) had not been regulated? Atmospheric Chemistry and Physics 9: 2113-2118. doi: 10.5194/acp-9-2113-2009
- Patterson L (2010) A chemical bond: Nick Barker, linking schools and universities in the UK. Science in School 15.
- Veneu-Lumb F, Costa M (2010) Using news in the science classroom. Science in School 15: 30-33.
Web References
- w1 – The British Antarctic Survey is responsible for the UK’s national scientific activities in Antarctica. See: www.antarctica.ac.uk
- w2 – The Advanced Global Atmospheric Gases Experiment, AGAGE, is a NASA-sponsored initiative that has been measuring the composition of the global atmosphere continuously since 1978, including CFCs and most non-CO2 greenhouse gases specified in the Kyoto protocol. To access their data and for more information, see: http://agage.eas.gatech.edu
Resources
- Sidney Chapman first derived the photolytic mechanism by which ozone is formed and degraded. See:
- Chapman S (1930) On ozone and atomic oxygen in the upper atmosphere.Philosophical Magazine Series 7 10(64): 369-383.
- Jonathan Shanklin, one of the scientists who discovered the ozone hole, published his reflections 25 years after the discovery:
- Shanklin J (2010) Reflections on the ozone hole. Nature 465: 34-35. doi: 10.1038/465034a
- Download the article free of charge here, or subscribe to Nature today: www.nature.com/subscribe
- Nature has also published a collection of articles that have advanced our understanding of the stratosphere and the ozone layer, or told the story of the discovery, some of which are freely available. See: www.nature.com/nature/focus/ozonehole
- NASA’s Ozone Hole Watch page offers historical ozone maps, ozone facts, an ozone-related multimedia gallery, a collection of teaching modules about ozone-related topics, and more. See: http://ozonewatch.gsfc.nasa.gov
- The University of Cambridge, UK, has compiled a virtual tour of the ozone hole, its history and science. The tour is available in English, French and German. See: www.atm.ch.cam.ac.uk/tour
- The 74 scientists who attended the panel review meeting for the 2002 ozone assessment in Les Diablerets, Switzerland, published 20 Questions and Answers about the Ozone Layer, including the contributions of cycles of solar activity and volcanic eruptions. See: www.gcrio.org or use the direct link: http://tinyurl.com/2wpvf9r
- Introduction to Atmospheric Chemistry by Harvard University’s Professor Daniel J Jacob, which is freely accessible as a PDF, contains a section on ozone, including the diagram ‘Chronology of the ozone hole’ (chapter 10.3.3). See: http://acmg.seas.harvard.edu/people or use the direct link: http://tinyurl.com/39vhy6a
- Ozzy Ozone is a United Nations Environment Programme website offering educational cartoons, games, a glossary and more – including downloadable education packs with student and teacher handbooks for both primary and secondary school. All material is available in English, French, and Spanish. See: www.ozzyozone.org
- The Ozone Depletion website by scientist and author Rod Jenkins contains comprehensive information: www.ozonedepletion.info
- The website of the United Nations Environment Programme’s OzonAction branch provides a large collection of data and information about ozone and the Montreal Protocol. See: www.unep.fr/ozonaction
- See also the pages of the United Nations Environment Programme Ozone secretariat, in English, French and Spanish: http://ozone.unep.org
- NASA offers two online videos of atmospheric developments over the Arctic, as measured with the Upper Atmosphere Research Satellite (UARS).
- You can watch the increasing concentration of chlorine nitrate in February / March 1993. See www.nasaimages.org or use the direct link: http://tinyurl.com/2w6wgh4
- This video shows the formation of polar stratospheric clouds. See www.nasaimages.org or use the direct link: http://tinyurl.com/33dfn6e
- In addition, NASA has published images of a season in the life of the ozone hole. See: www.nasa.gov/vision/earth/lookingatearth/25TOMSAGU.html
Review
The ozone hole is a topical global issue, and you will find this article really helpful to get into the subject. The chemical processes involved are described in full detail. In chemistry lessons, the article can be used to teach atomic structure and chemical bonds, free radicals, catalytic cycles, and the influence of light and temperature on chemical reactions.
For the earth science classroom, the article would fit in the context of the following topics:
- Atmospheric influence on climate
- Biological processes occurring on Earth’s surface and affecting marine organisms
- Earth’s morphology and the distribution of mountain ranges on Earth’s surface
- The changing of the seasons, Earth’s axial tilt and rotation.
There is the opportunity for interdisciplinary work linking chemistry and earth sciences. Possible topics include:
- The geographical distribution of the organisms that produce chemicals that are active in natural catalytic cycles. Where do those organisms live? Which chemical substances do they produce?
- The chemical composition of the atmosphere and its influence on the climate. Which gases is the atmosphere composed of? In which way do the atmosphere’s properties determine climatic conditions on Earth’s surface, and how does this differ on other planets?
The article could also form the basis of a lesson on how science is reported in the media. Students could compare this article to those in the general press: do they provide a balanced view of the question, mentioning both chemical and natural components leading to the formation of the ozone hole? Do they minimise or overemphasise the phenomenon as a whole? Why – due to journalists’ ignorance, political strategy or both? For further ideas on using news in the science classroom, see Veneu-Lumb & Costa (2010).
Finally, the text is suitable as the basis of a comprehension exercise, too. Possible questions are:
- Why is this topic a much debated question nowadays?
- What is the role of natural factors in the growth of the ozone hole? What about human factors?
Teresa Celestino, Italy