Accelerating the pace of science: interview with CERN’s Rolf Heuer Understand article
CERN’s director general tells the story behind the Higgs boson – and describes the next steps.
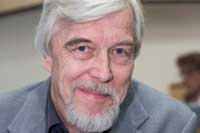
Image courtesy of CERN
CERN is not just the world’s largest particle physics laboratory. As its director general, Rolf Heuer, explains, “CERN is a role model, demonstrating that science can bridge cultures and nations. Science is a universal language and this is what we speak at CERN.”

houses the LHC. To allow for
the necessary measurements
on the new particle, the LHC
will run until February 2013.
Professor Heuer explains:
“Imagine you see someone a
long way away who looks
like your best friend. To be
certain that it is not actually
his or her twin, you need to
wait until you’ re close
enough. That takes time.”
Image courtesy of CERN
CERN also unites people in other ways. “As a young summer student, you can find yourself having lunch next to a Nobel Prize winner. And everyone, from the canteen workers to the senior management – we all identify ourselves with CERN, sharing a desire to increase human knowledge. We all do our bit towards that goal, leaving political, cultural and educational differences outside the campus.”
This undoubtedly makes CERN a very special place to work, but what makes it unique is its particle accelerator, the Large Hadron Collider (LHC). First used in 2008, the LHC is the world’s largest particle accelerator, its 27 km tunnel forming a ring beneath the French-Swiss border. As Professor Heuer explains, “It’s also one of the coldest places in the Universe, cooled to 1.9 K with superfluid helium. Even outer space is warmer, at 2.7 K.
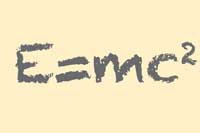
tells us that to create the
very heavy particles that
existed a fraction of a
second after the Big Bang,
the LHC needs to collide
particles at very high
energies.
Image courtesy of Nicola Graf
“Simultaneously, it’s one of the hottest places in our galaxy because when we collide protons in the LHC, we produce temperatures that are much, much higher than those at the centre of the Sun.” Professor Heuer describes the collision of two protons at close to the speed of light as being like two mosquitoes colliding in mid-flight.
“The key difference is that these protons are tiny, tiny, tiny particles, so their energy density – the energy of the protons divided by their volume – is huge, and it is this energy density that brings us close to the Big Bang.”
As detailed in two previous Science in School articles (Landua & Rau, 2008; Landua, 2008), these enormously energetic collisions can create very heavy particles, the sort of particles that were formed in the extremely energetic conditions a fraction of a second after the Big Bang. These are particles so massive that they have not been created since then (remember that Einstein’s law E=mc2 tells us that to create a very heavy particle, we need a large amount of energy).
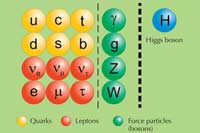
describes the fundamental
particles from which we, and
every visible thing in the
Universe, are made, and the
forces acting between them.
Click on image to enlarge.
Image courtesy of CERN
As Professor Heuer and I talk, extremely distant history has just been re-enacted: on 4 July 2012, CERN announced the detection by the LHC of a particle that is ‘consistent with the Higgs boson’, last created about 10-12 seconds after the Big Bang. This is momentous news. “We’ve been looking for this particle for 40 years. I’m not sure if I’ve digested the news yet, but I think this might be one of the biggest discoveries of recent decades,” he tells me.
If the newly detected particle is indeed the Higgs boson, this discovery will validate the standard model of particle physics. The standard model (figure 1) describes the fundamental particles from which we and every visible thing in the Universe are made, and the forces acting between them. And as Professor Heuer explains, “The Higgs boson was the missing cornerstone of the standard model.”
The discovery would also explain why particles – and thus matter – have mass. The search for the Higgs boson began in the 1960s, when a group of physicists, including Peter Higgs, postulated what is now known as the Higgs field. Immediately after the Big Bang, they believed, particles had no mass but rapidly acquired it by interacting with this field; the more the particles interacted with the Higgs field, the more massive they became.
“Imagine that the Higgs field is a party of journalists, equally distributed in the room,” says Professor Heuer. “I can pass through the room mass-less – with the velocity of light – because they don’t know me. If someone well known enters, the journalists cluster around that person: the person’s velocity is limited and he or she acquires mass. The better known that person is, the more the journalists cluster around, and the more massive that person becomes. This is how a particle acquires mass from the Higgs field.” See figure 2.
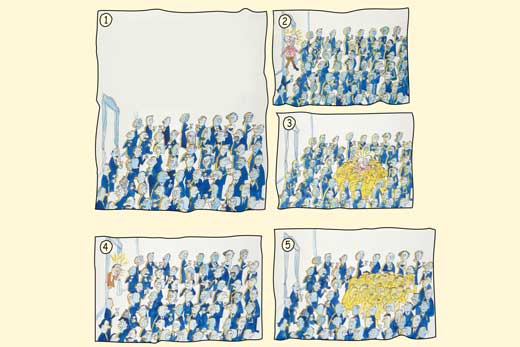
1. To understand the Higgs mechanism, imagine that a room full of
journalists quietly chattering is like space filled only with the Higgs field.
2. A well-known scientist, Albert Einstein, walks in, creating a disturbance as he
moves across the room, and attracting a cluster of admirers with each step.
3. This increases his resistance to movements – in other words, he acquires
mass, just like a particle moving through the Higgs field.
4. If a rumour crosses the room…
5. …it creates the same kind of clustering. But this time among the journalists
themselves. In this analogy, these clusters are the Higgs particles.
Image courtesy of CERN
But where does the Higgs boson come in? By definition, bosons are particles with an internal angular momentum – known as spin – corresponding to an integer multiple of the Planck constant (e.g. 0, 1 or 2). Some bosons are force particles, through which matter particles interact with each other. For example, a photon is a boson that carries the electromagnetic force; a graviton is a boson that carries the gravitational force. The Higgs boson, however, is postulated to be different: it is the result of the Higgs field interacting with itself (figure 2). “Suppose I open the door to the journalists’ party and whisper a rumour into the room. The journalists will be curious – ‘what did he say?’ That’s the journalists interacting among themselves – or the self-interaction of the Higgs field: that is a Higgs boson.”

by Professor Heuer as “very
moved by the announcement.
He said that if we had
excluded the possibility of
the Higgs boson’s existence,
he would no longer have
understood particle physics.”
Image courtesy of Murdo
MacLeod
The only problem with the Higgs boson was that nobody knew if it actually existed. Over the years, larger and larger particle accelerators have been built to look for it, capable of colliding particles with higher and higher energies. This enabled physicists to create more and more massive particles, but there was still no sign of the Higgs boson. Did it not exist after all, or did it just require a still more powerful accelerator to detect it? The new particle may well have answered that question.
So how did the CERN scientists actually detect this new particle?
The signal they were looking for was the decay of the Higgs boson. However, the scientists needed to be able to distinguish the decay pattern of the Higgs boson from the decay signals of the many, many other particles created in the LHC. As Professor Heuer jokes, “It’s like looking for one type of snowflake by taking photos of it against a background of a snowstorm. Very difficult.”
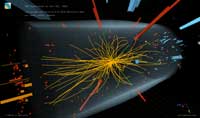
of data from one of the many
collisions that was used to
search for the Higgs boson.
“It’s not looking for a needle
in a haystack: this is looking
for a needle in millions of
haystacks, with the slight
problem that the haystacks
also consist of needles and
we have to find one needle
with a very slight difference
from in among many, many
other needles,” Professor
Heuer explains.
Image courtesy of CERN
One promising signal to look for was the decay of the Higgs boson into two photons, specifically two high-energy photons. When two photons are detected originating from the same spot, they may be the result of a Higgs boson decaying. On the other hand, they may be part of the background noise of other particle collisions and decays occurring in the LHC. So how do the scientists distinguish the two?
The answer is that they cannot, in any one case, tell whether the photons originate from a Higgs boson or from the decay of some other particle, but they can use statistical analysis to test whether the number of decays detected is what they would expect. For this, they construct a null hypothesis – in this case, that the Higgs particle does not exist – and predict what they would find if the null hypothesis were true. If more decays were detected than expected, this would indicate the existence of the Higgs boson.
This was precisely what two of the LHC experiments, ATLAS and CMS, found in July 2011: above the smooth curve of the expected results, there was a deviation representing more decays than expected. Importantly, both experiments found this deviation at the same point – representing the decay of particles with a mass of 126 GeV – and the deviation had the same magnitude – representing the same number of ‘extra’ decays in the accelerator. The question was, were these deviations statistically significant? For new discoveries in particle physics, the bar for statistical significance is set very highw1: at five sigma, which represents about a one in 3.5 million chance of detecting more decays than expected by chance alone, even if the null hypothesis were true.
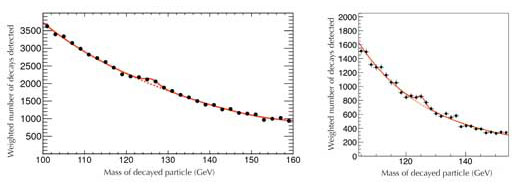
showing the (weighted) number of decays detected at each particle mass.
The gap between the dotted and solid red lines shows the deviation from
the expected results, representing the decay of the new particle.
Click on image to enlarge.
Image courtesy of CERN
The initial data in July 2011 certainly looked promising, but offered nowhere near this level of certainty. Over the following year, however, the two LHC experiments gathered more and more data, all pointing in the same direction: there were more two-photon events with a mass of 126 GeV than would be expected if there were no Higgs boson. Finally, on 4 July 2012, the five-sigma threshold was crossed and the CERN scientists were confident enough to announce to the world that they had indeed detected ‘a particle consistent with the Higgs boson’.
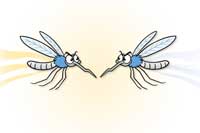
the collision of particles in
the LHC as being like two
mosquitoes colliding in
mid-flight.
Image courtesy of ARTPUPPY /
iStockphoto
For most of us, despite previous tentative statements from CERN, this announcement came quite unexpectedly. For Professor Heuer, in contrast, the excitement had been building up over months, but the step-by-step revelation of the discovery did not reduce its impact one bit. “The discovery is the most exciting moment of my career, because we are writing a little bit of history.”
So how much do we actually know about this new particle?
“We know it’s a new particle and we know it’s a boson. It’s the heaviest boson ever found, and it looks like the Higgs boson. However, scientists can be very cautious. As a layman, I would say ‘we’ve found the Higgs boson’. As a scientist, I have to ask ‘what have we found?’”
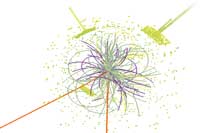
The next step, therefore, is to measure the properties of this particle, including its spin. All previously known bosons are particles with spin 1, for example photons. They are associated with vector fields: the electromagnetic field, for instance, is a vector field that has both a direction and a strength. As a result, the photon is moved in a particular direction: it has spin. The Higgs boson, however, is postulated to be different – it is associated with a scalar field, the Higgs field, and that means it has spin 0.
“If you swim in a river, the force that the water exerts on you will depend on which direction you swim in. That would be a vector field. If, in contrast, you are in a swimming pool, the force that the water exerts on you will be the same whichever direction you swim in. That’s a scalar field.”
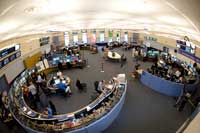
Image courtesy of CERN
It’s also important to measure the new particle’s mass more accurately. “Instead of being the Higgs boson, it could be a Higgs boson. The standard model predicts only one, but supersymmetry – an extension of the standard model (as explained in Landua & Rau, 2008) – predicts at least five. And the lowest-mass Higgs boson predicted by supersymmetry is very similar in mass to that predicted by the standard model.
“That makes it difficult to distinguish the two; we need more measurements.” To this end, the LHC will collect data from as many collision events as possible before February 2013, when it will be closed until the end of 2014 to refit it for still higher energy collisions, enabling it to create and detect even heavier particles.
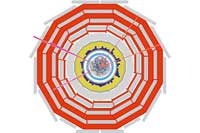
So if the measurements over the next few months show the new particle to be the (or a) Higgs boson, then the standard model would be validated, proving the existence of the Higgs field, and thus confirming the mechanism by which particles acquire mass. But what if the newly discovered boson turns out not to be a Higgs boson? “If it’s slightly different to what we expected, it could introduce physics beyond the standard model.”
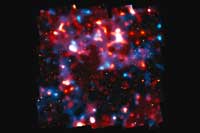
distribution of ‘normal’
matter, traced via hot gas,
seen by the space telescope
XMM- Newton (in red) and
stars and galaxies observed
with the Hubble Space
Telescope (in grey), to the
distribution of the invisible
dark matter (in blue), which
has been inferred from the
gravitational lensing effect.
The map demonstrates how
‘normal’ matter across the
Universe follows the
structure of an underlying
‘scaffolding’ of dark matter.
Image courtesy of NASA / ESA /
R Massey (California Institute of
Technology)
Whatever the outcome of the measurements on the new particle, once the LHC is reopened, it will turn its focus beyond the standard model, which describes only the visible Universe – thought to be no more than 4-5% of the total energy balance of the Universe. As Professor Heuer points out, “The standard model leaves many questions open. For example, it doesn’t tell us what happened to the antimatter that existed at the beginning of the Universe, nor does it tell us in how many space or time dimensions we are living. And it casts not the faintest light on what dark matter or dark energy is.”
Of the dark 95% of the Universe that is not addressed by the standard model, 25% is thought to be dark matter. “When we compare that to the 5% that comprises the visible Universe, it’s obvious that dark matter must have played a dominant role in shaping the early Universe. Astronomers can tell us how it has shaped the Universe, but only particle accelerators are likely to be able to produce dark matter in the laboratory and help us understand exactly what it is. Is dark matter composed of a single kind of particle or is it rich and varied like the normal world?” One potential answer involves supersymmetry, and after refurbishment, the LHC will be powerful enough to create and detect some of the very massive particles that supersymmetry would predict.
The other three quarters of the dark Universe is dark energy, thought to drive the Universe apart. Professor Heuer believes that the LHC and its investigation of the Higgs boson could be important here too.
“The Higgs field is scalar, as is dark energy. They are not the same, but studying the Higgs field might tell us a lot about dark energy.”
In short, “So far, we know very little about dark matter and we know essentially nothing about dark energy, but I think that, with the LHC, we are about to enter the dark Universe.”
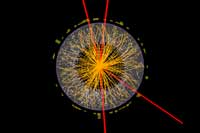
Throughout our interview, it’s been obvious just how much Professor Heuer relishes bringing physics alive for non-specialists. He’s clearly very good at it, too: “I gave a public lecture in the Royal Society in London in which I presented the LHC, the science around it and the fascination of the dark Universe. A day later, I received an email from a 14-year-old boy who wrote that he was doing very well in maths and physics and that he wanted to start work at CERN in 2018.”
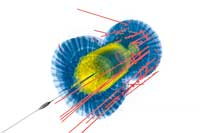
The difficulty, as Professor Heuer acknowledges, is not rousing young people’s enthusiasm for science, but maintaining it. To this end, he emphasises the importance of “explaining new developments and important topics in science, for example using Science in School”. Professor Heuer is clearly a fan.
As we close our interview, I ask if Professor Heuer has any further advice for our readers. “Enthusing students within the current school curricula can be very difficult – if you start with 19th-century mechanics, you will lose 99% of them immediately. Introducing modern science, however, can really help.” Fortunately, he is convinced that a lot can be explained without mathematics.
“For example, I explained the Higgs mechanism by talking about journalists. Of course, to fully understand it and explain it, your students would need to use mathematics, but they can always look that up. What they need to understand is the logic.”
References
- Landua, R (2008) The LHC: a look inside. Science in School 10: 34-45.
- Landua R, Rau M (2008) The LHC – a step closer to the Big Bang. Science in School 10: 26-33.
Web References
- w1 – To find out more about the statistical analysis, see ‘5 sigma – what’s that?’ in the Scientific American blog.
Resources
- To learn more about the LHC shut-down, planned for 2013-14, see:
- Brewster S (2012) Scientists already planning for LHC long shutdown. Symmetry September 2012.
- To learn more about the research leading to the discovery of the new particle, see:
- Baggott J (2012) Higgs: The invention and discovery of the ‘God Particle’. Oxford, UK: Oxford University Press. ISBN: 9780199603497
- For an explanation of the LHC in layperson’s terms, see:
- Ginter P, Franzobel, Heuer RD (2011) LHC: Large Hadron Collider. Paris, France: UNESCO. ISBN: 9783901753282
- Watch Rolf Heuer’s lecture ‘The search for a deeper understanding of our Universe at the Large Hadron Collider: the world’s largest particle accelerator’, given at the Euroscience Open Forum in Dublin, on 14 July 2012, just 10 days after the announcement that a boson compatible with the Higgs boson had been discovered at CERN.
- To learn more about the next generation of particle accelerators that will study the Higgs boson in more detail, see:
- Chalmers M (2012) After the Higgs: the new particle landscape. Nature 488: 572-575. doi: 10.1038/488572a
- Download the article free of charge here, or subscribe to Nature today.
- Read two interviews with scientists working on dark matter and dark energy:
- Boffin H (2008) “Intelligence is of secondary importance in research”. Science in School 10: 14-19.
- Warmbein B (2007) Making dark matter a little brighter. Science in School 5: 78-80.
Institutions
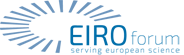