Good vibrations: how to catch a gravitational wave Understand article
Gravitational waves are among the most subtle messengers that reach us across the cosmos. But how can their infinitesimal effects be detected?
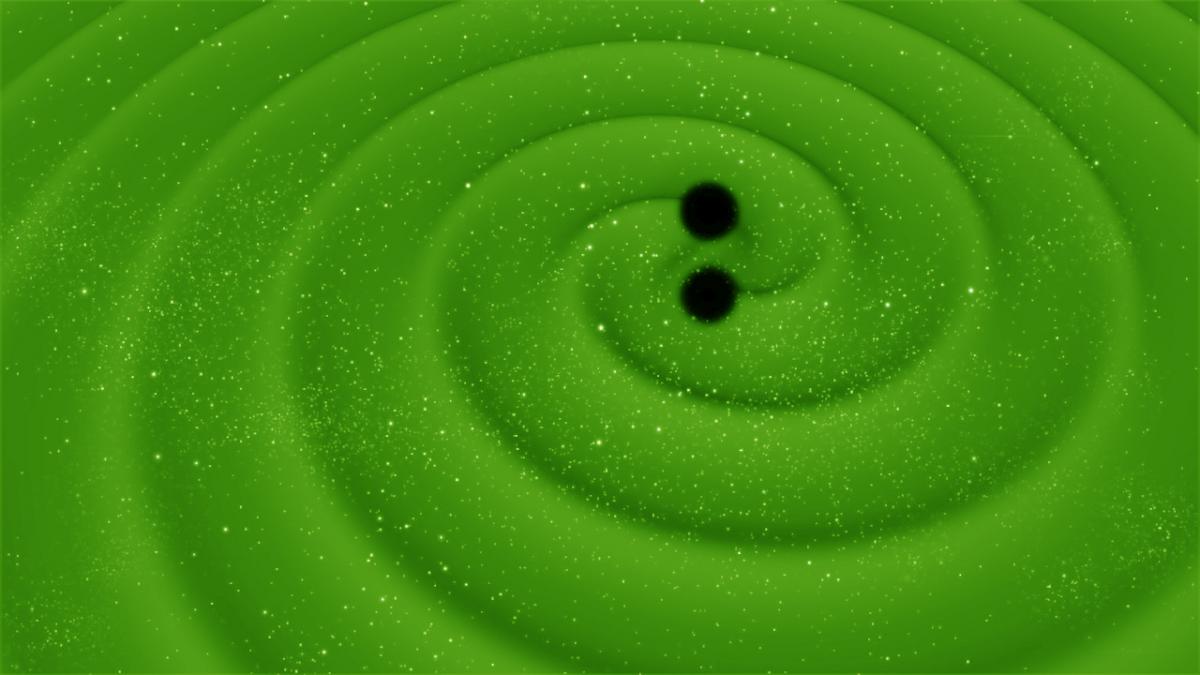
black holes as they spiral
towards each other before
merging, releasing
gravitational waves
Image courtesy of ESA / C
Carreau
In 2015, the fantastically faint signals from gravitational waves crossing the cosmos were finally detected. Predicted a century ago by Albert Einstein, this first detection of gravitational waves marked the culmination of decades of experimental and theoretical work – and also the beginning of an exciting new era in cosmology.
So what are gravitational waves, and why was detecting them such a huge challenge? Gravitational waves are ripples in space-time caused when a mass accelerates. In effect, they are the gravitational equivalent of electromagnetic waves – and, like these, they travel at the speed of light.
The reason these elusive signals are so hard to detect is because their effects are so very, very slight: just a tiny distortion in space-time, even when the event producing them is as vast as the collision of two (distant) black holes, as was the case with the 2015 detections. This distortion means that the distance between two points on Earth will be stretched or squeezed by an absolutely tiny fraction when a gravitational wave passes by. And here, ‘tiny’ means by a factor of 10-21 (one thousand billion billionth) – roughly the diameter of an atom compared to the distance between Earth and the Sun: certainly quite a challenge to measure!
Since the early 1960s, physicists, engineers and technicians from all over the world have risen to this challenge, resulting in a handful of giant instruments dedicated to detecting gravitational waves, including LIGOw1, Virgow2 and GEO600w3. In this article, we focus on the Virgo instrument (located in Italy), but the concepts apply equally to the other detectors – all of which are part of an international network that is much more powerful than any individual detector alone. In fact, the analysis of the 2015 data comprising the first detections was carried out jointly by LIGO and Virgo scientists, working in collaboration.
Measuring with light
Virgo’s design is based on a device called a Michelson interferometer, which itself has a remarkable pedigree: it was first used in 1887 by physicists Albert Michelson and Edward Morley in a famous experiment to look for variations in the speed of light caused by the hypothetical ether (figure 1).
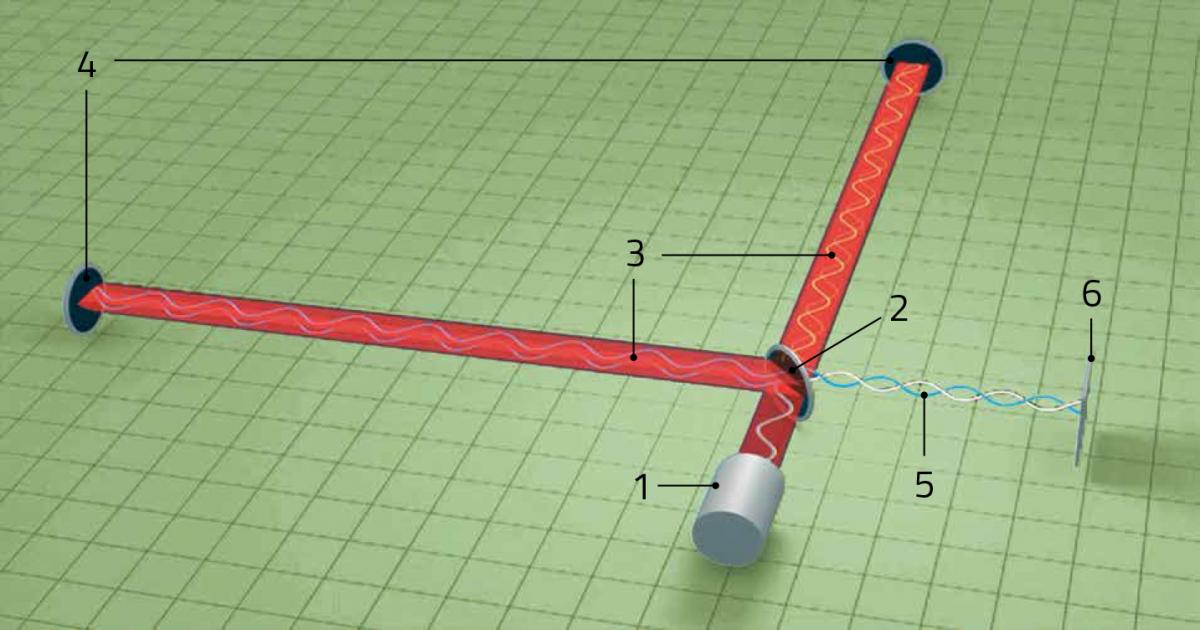
Image courtesy of LIGO / T Pyle
Here, light from a single source is split into two beams that travel out along perpendicular paths (or ‘arms’) and are then reflected back by mirrors so that they ultimately recombine. If there is a change in the length of one beam’s path (as would be caused by a passing gravitational wave), this will very slightly change its travel time, and thus also cause a phase shift of one beam relative to the other. This phase shift affects how the two beams will interact when they meet on their return, which in turn affects the power measured at the detector output.
But even with this classic design combined with modern technology, the experimental challenges of detecting gravitational waves are very considerable.
Virgo: overcoming the challenges
In Virgo, the basic design of the Michelson interferometer has been made much more complex – and bigger – because of the extreme stability and precision needed.
Long arms
Each arm of the Virgo detector is 3 km long. This large size is needed because the extremely small change in the beams’ travel time, caused by a gravitational wave, increases with the arm length. Lengths beyond 3 km are not really feasible; one reason is that the Earth’s curvature would then become a factor in building perfectly straight arms.
To prevent interactions between the beams’ photons and gas molecules, the interior of the arms is evacuated to about one thousandth of a billionth (10-12) of atmospheric pressure, similar to the pressure in space at the altitude of the International Space Station. This makes the Virgo tubes the largest ultrahigh-vacuum volume in Europe (see figure 2). At the end of each arm, the wall of the tube is cooled to cryogenic temperatures by liquid nitrogen to trap residual molecules (e.g. water).
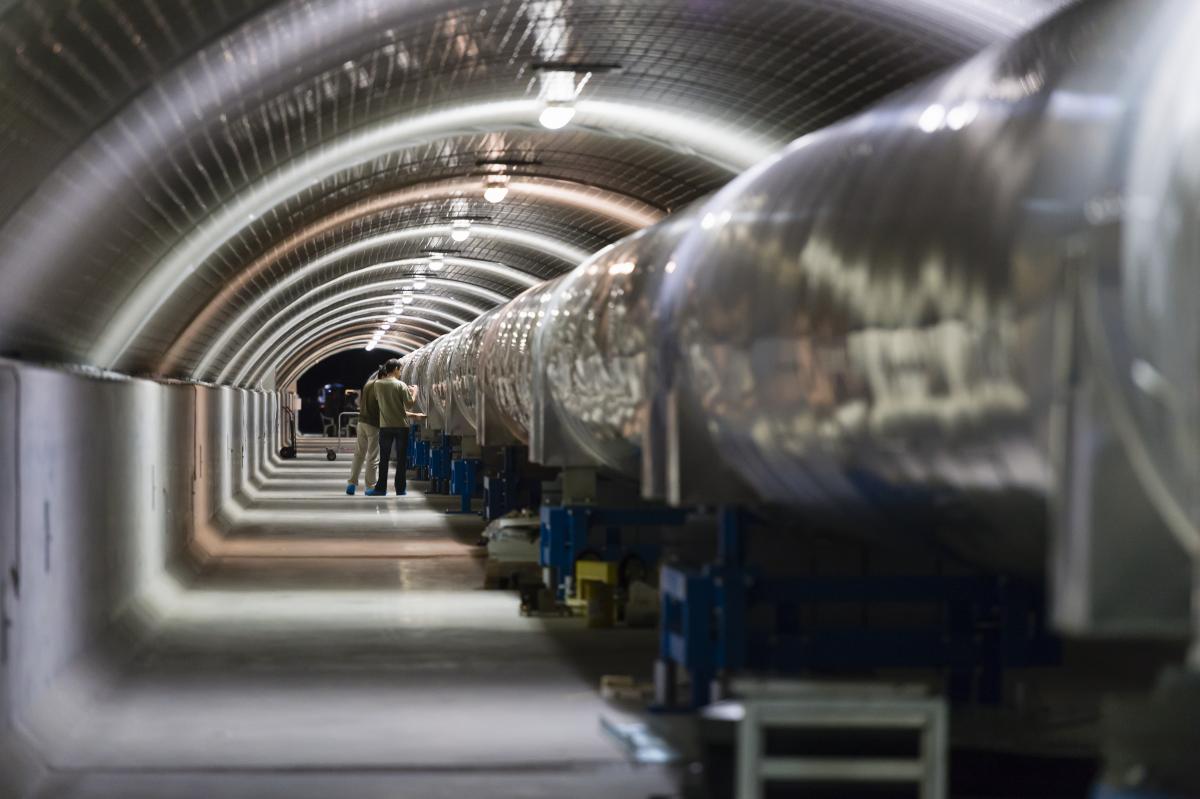
Image courtesy of Cyril Fresillon / Virgo / CNRS Photothèque
Mirrors, mirrors
The mirrors in Virgo are a key component of the detector. They are made with the utmost precision: their surfaces are polished to be perfectly flat (to the nearest nanometer), and special coatings optimise the way the mirrors reflect and transmit light, keeping losses from the beams to a minimum (around a few parts per million). The mirror arrangement is much more complicated than in a simple Michelson interferometer, with mirrors used to form additional ‘optical cavities’ through which the beam travels, or to ‘clean’ the laser beam (see figure 3).
Virgo’s mirrors also play a trick to make the beam paths seem longer than they are: a device called a Fabry-Perot optical cavity installed in each arm increases the path length by a factor of around 300, increasing the beams’ travel time – and thus the sensitivity of the whole detector – by a similar factor.
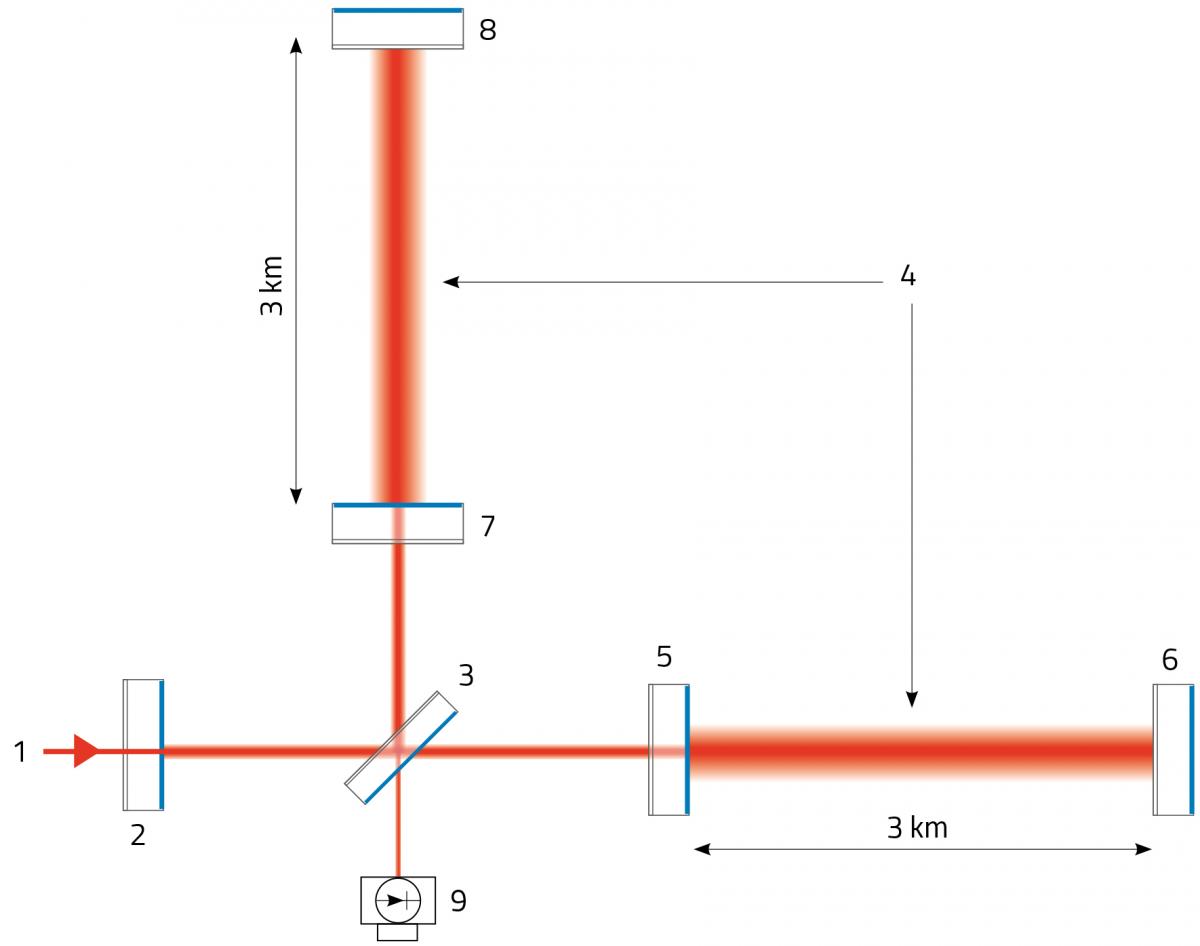
Image courtesy of The Virgo Collaboration
Vibration isolation
Virgo needs to be sensitive to the very small changes in the lengths of the beam paths induced by gravitational waves, so it needs to be isolated as much as possible from other disturbances in the environment – human activities, wind, storms, and so on. While the detector’s design aims to shield it from such disturbances, one of the biggest problems is that the mirrors (which reflect the laser beams) are attached to the ground, which moves all the time – too slightly for us to feel, but by far more than the changes due to gravitational waves.
This means that the mirrors must be isolated from the ground – a feat achieved in Virgo by suspending each mirror at the end of a chain of pendulums called ‘super-attenuators’, which make its suspended mirrors some of the most motionless objects on the planet (see figure 4). But how does suspending an object isolate it from vibrations?
Every simple pendulum has its own natural or ‘resonance’ frequency – the frequency at which it will swing if just given a push. If we shake the top of the pendulum at a frequency lower than the resonance frequency, the end of the pendulum will move. But if the input motion is at a frequency higher than the resonance, the lower end will remain almost still. In Virgo, the pendulums supporting the mirrors have resonance frequencies that are as low as possible (a few hertz). This means that they are essentially undisturbed by movements at higher frequencies, allowing gravitational waves of frequencies above a few tens of hertz to be detected.
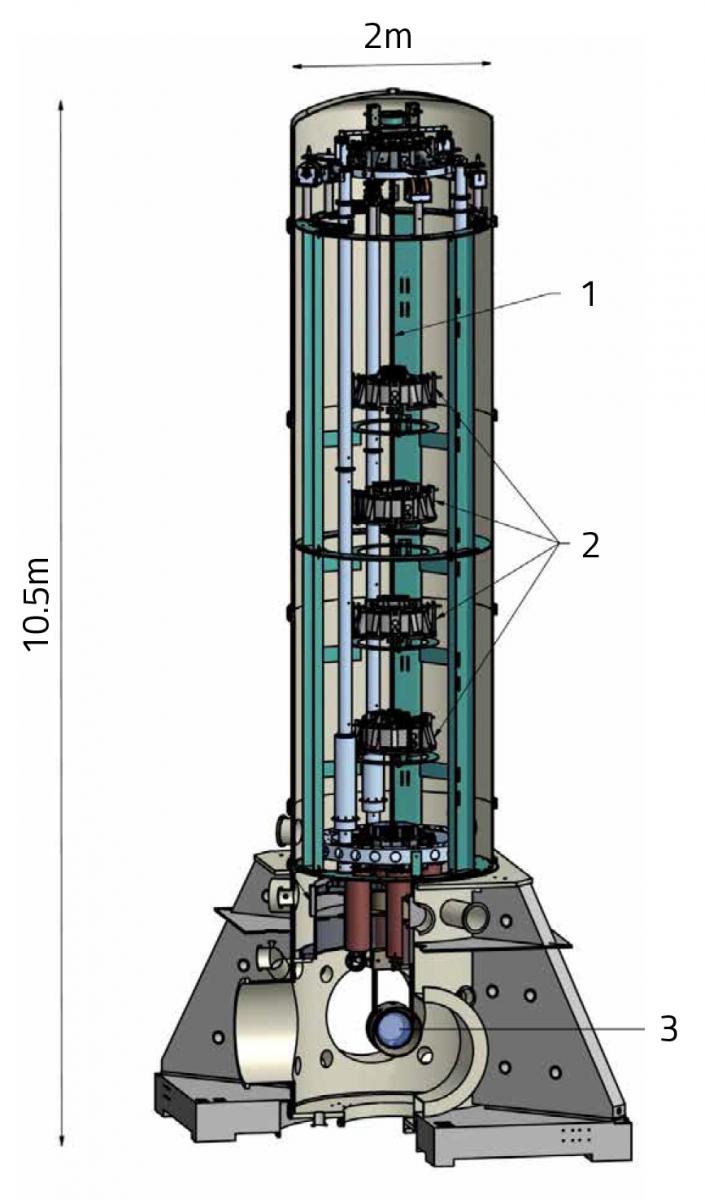
1: pendulum wire; 2: pendulum chain; 3: mirror
Image courtesy of The Virgo Collaboration
Maintaining precision
To be sensitive to gravitational waves whenever one might arrive, Virgo must be constantly maintained in a very precisely controlled working state. For example, the laser beam (an infrared laser with a wavelength of 1064 nm) must be kept ultra-stable to maintain a constant power level at the detector output. The laser’s frequency must also be stabilised so that it varies less than one part in 1014.
Probes located all along the Virgo apparatus continuously monitor its status and allow optical cavity lengths to be controlled at the femtometre (10-15 m) level, while mirror-angle misalignments are kept to within a few nanoradians (less than a millionth of a degree). In addition, thousands of probes constantly monitor Virgo’s environment and status, providing data to be checked as soon as a potential gravitational wave signal is observed.
Finding out more
There is much more to explain about the Virgo experiment – for example, how is the data analysed to find out if a gravitational wave has really been detected? If you would like to know more, please visit our websitew2 or read the recent article in Science in School that describes how gravitational waves were first detected (Kwon, 2017) – and the discoveries that this new ability could bring to astrophysics.
Acknowledgement
The author would like to thank Dan Hoak (European Gravitational Observatory) for his help in preparing this article.
References
- For an earlier article in Science in School about detecting gravitational waves, see:
- Kwon D (2017) Turning on the cosmic microphone. Science in School 39: 8-11.
Web References
- w1 – The Laser Interferometer Gravitational-Wave Observatory (LIGO) is based in California, USA.
- w2 – Find out more information on Virgo from the Virgo website.
- w3 – GEO600 is a ground-based interferometric gravitational wave detector located near Hannover, Germany.
Resources
- To find out how the Virgo detector works, watch this short animation.
- Watch the press conference announcing the first gravitational wave detections.
- Learn more about LIGO Open Science Center, including data from the 2015 detections.
- The LIGO-Virgo collaboration has its own YouTube channel featuring talks, lectures and explainer videos.
- Find out more about gravitation and gravitational waves from an animation and comic in several languages, made by PhD Comics.
Review
This article describes the function of gravitational wave detectors – huge but very sensitive machines that students may find very interesting. The details of how these machines work, and the problems that had to be solved to achieve their high sensitivity, are described in a very understandable way by the author, who is a gravitational wave scientist.
Comprehension questions could include:
- What are gravitational waves?
- Why is it so difficult to detect gravitational waves?
- How do gravitational detectors work?
- Gravitational wave detectors are very sensitive sensors. Why are these machines so large?
- Building gravitational wave detectors is a real challenge. Describe the major difficulties and how they can be solved.
Gerd Vogt, Higher Secondary School for Environment and Economics, Austria