Programmable metallisation cells: the race for miniaturisation Understand article
Gabriel Cuello from the Institut Laue-Langevin (ILL) in Grenoble, France, introduces a new type of digital memory that may revolutionise our USB sticks.
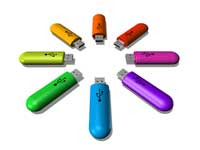
The wish to download, store and carry music and videos from the Internet has led to massive and ever-increasing mobile storage needs. All this information is stored in just two distinct states of a bit: 0 or 1. In the flash memory we all use in USB sticks and memory cards (for example, in digital cameras), these are two distinct voltage or current levels allowed by a semiconductor-based integrated circuit, with millions of tiny transistors or capacitors which can be electrically erased and reprogrammed, storing information as electrical charge.
Importantly, the stored information is retained even when the device isn’t powered. But flash memory has a drawback: its storage capacity is usually limited to a few gigabytes. For higher storage capacities, today, external hard drives are used, but unlike flash memory they require an external power supply, so just reducing their size is not ideal. Yet if the storage capacity of USB sticks could be extended to several terabytes, this could be the way forward. A new kind of memory device based on programmable metallisation cells (PMCs) could be the solution.
PMCs not only use less energy than flash memory and have faster access times (a few nanoseconds) due to their nanometric size, they can also be made physically flexible, have a higher storage capacity and longer lifetimes (their data can be overwritten 1010 times before the data integrity starts to wear, compared to 105 write-erase cycles in flash memory), and can withstand elevated temperatures for more than 10 years (Kozicki et al., 2005).
Essentially, PMCs store information as two different states: high and low electrical resistance. This is achieved through a conducting nanopath of metal atoms (silver or copper), which forms itself through ionic redistribution and electrochemical processes. When an electrical potential is applied in one direction, it remains intact until a potential is applied in the opposite direction, at which point the nanopath dissolves again – this is the ‘programmable metallisation’. When the nanopath is present, electricity can flow through the PMC with little resistance: this is state ‘1’. Without the nanopath, resistance is high: this is state ‘0’. The complete cycle is schematically represented in Figure 1 below.
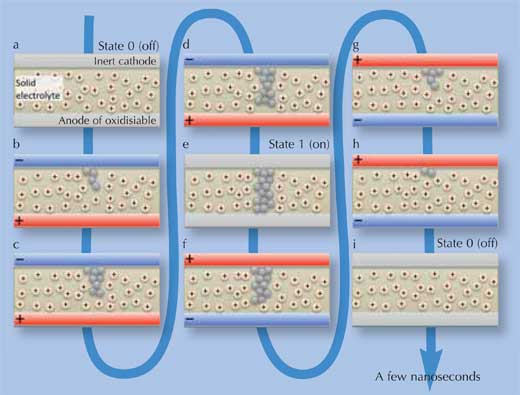
Image courtesy of Gabriel Cuello
How does this work? A PMC has three main components: a solid electrolyte film (an ion-containing medium, commonly silver germanium selenide (AgGeSe), disilver selenide (Ag2Se), copper germanium selenide (CuGeS) or dicopper sulphide (Cu2S)), sandwiched between two electrodes, no thicker than 100 nm in total. The anode is an oxidisable layer of metal (silver or copper – the same as the ions in the medium in between), which can release these ions to the central medium. The cathode is a thin layer of a metal such as tungsten (however, not a source of ions, therefore ‘inert’), as shown in Figure 1(a).
For them to function effectively, the important feature of PMCs is the characteristics of the central solid electrolyte. Such electrolytes are usually amorphous, and their atoms have an intermediate mobility between that of atoms in liquids and those in crystals. Unlike in a liquid electrolyte (such as those used in common batteries), only the positively charged ions are mobile, while the negatively charged counter-ions are fixed. This is essential for the formation of the conducting nanopath from electrodeposited cations.
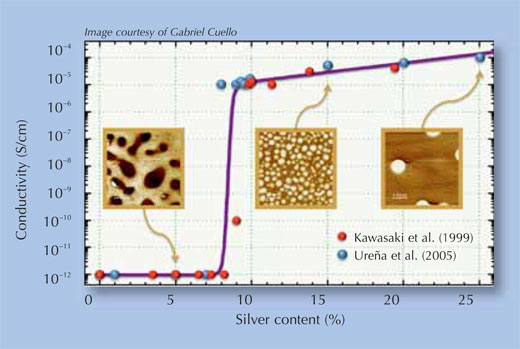
Image courtesy of Gabriel Cuello
Many inorganic and organic (including polymeric) materials can conduct ions, and therefore act as solid electrolytes, but only the chalcogens (Group 16 in the periodic table of elements) are interesting in the context of PMCs, because they have the right electrical properties. Combining elements that make thermally stable compounds (such as oxygen, sulphur and selenium) with copper or silver yields binary electrolytes such as disilver selenide and dicopper sulphide. The conductivity of the material can be improved even further by using ternary electrolytes: chalcogens are combined with other elements such as germanium, to create a glass (an amorphous material in which the atoms are not in a strict order), in which copper or silver can be dissolved. The conductivity is further determined by the concentration of the metal ions in the electrolyte, since nanopaths don not form in the entire cell but only in those regions where the ion concentration is especially high (see Figure 2). Therefore, electrolytes with different metal concentrations are studied to find the ideal composition.
Although ternary electrolytes have better characteristics for use in PMCs, interactions between three types of ions are more difficult to study than those between just two types, which is why, so far, the characteristics of binary solid electrolytes are better known.
Research at ILL
To learn more about these ternary electrolytes, my colleagues and I at ILLw1 have studied AgGeSe glasses with different silver contents. To understand the electrical properties of a material, it is important to first understand its structure. Hence, we wanted to determine the local order of the atoms grouped around the silver atoms. The order of the atoms in the glass as a whole is random, but if you look locally, it is possible to predict the probability of finding a certain type of atom within a specific (close) range. The further you get away from this silver atom, however, the less certain you can be about the atoms you will find there.
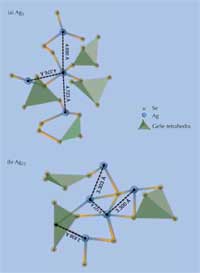
Agx(Ge0.25Se0.75)100-x containing
(a) x = 5 and (b) x =
25 (percent silver). Characteristic
bond distances between silver
atoms are shown in Ångström.
Germanium atoms are located in
the center of each tetrahedron.
Click to enlarge image
Image courtesy of Gabriel Cuello
We analysed the glasses using neutron diffraction, a technique regularly used at ILL, in which materials are analysed by the way they diffract a beam of neutrons, and which, importantly, does not necessarily require a regular order of the atoms (see Cuello, 2008). Not only the nuclei of different elements but also the nuclei of different isotopes of an element scatter neutrons with different power. Taking advantage of this, a given type of atom under study can be substituted by an isotope, which does not change the structure of the material. In this case, we were interested in the silver atoms, so we replaced natAg with 107Ag and 109Ag, the two stable isotopes that comprise natural silver (natAg). Comparing the diffraction patterns obtained from natAgGeSe, 107AgGeSe and 109AgGeSe, we were able to identify which parts of the diffraction pattern were related to the silver atoms.
Using a neutron beam of short wavelength allows the determination of the short-range order – the probability of finding a specific type of atom at a given (short) distance from another. To calculate the correlations between three different species of atoms (silver, germanium and selenium), a ternary system, you would ideally do six independent measurements. Three measurements, however, as were performed here, provide a good approximation (this is why two different silver isotopes were used), which can then be complemented using computer simulations of molecular dynamics.
This combination of neutron diffraction experiments and computer modelling allows us to propose a local structural image of the ternary electrolyte (see Figure 3). There is a clear phase separation between the conducting (silver-rich) regions and the non-conducting ones (silver-poor) (Piarristeguy et al., 2008), which is important for the distinct establishment of the low- and high-resistance states. In the case of 25% atomic silver dissolved in germanium triselenide (GeSe3), i.e. Ag25(Ge0.25Se0.75)75, there is a continuous glassy digermanium triselenide Ge2Se3 backbone and a dispersed nanoscale disilver selenide (Ag2Se) phase. The metal-rich Ag2Se phase is both an ion and an electron conductor, important for the establishment of a low-resistance state with a nanofilament through which a current can flow. The Ge2Se3 backbone, by contrast, which separates each of these conducting regions, is a good dielectric (a non-conductor material which becomes a conductor if you apply a strong enough electric potential), so the overall resistance of the material prior to electrodeposition, i.e. before a forward current is applied to form a conducting nanofilament, is high.
In AgGeSe containing only 5% silver, although the basic phase separation is the same, the distances between silver atoms are higher (see Figure 3), which explains the lower conductivity of the material.
There is still much to learn about the exact process that creates these nanopaths, but this is a promising new technology which could certainly improve the performance of digital memory devices in the near future. However, nano-ionic memory such as PMCs is only one of many possibilities being explored, and it remains to be seen which one of them will eventually make its way into our homes.
References
- Cuello GJ (2008) Structure factor determination of amorphous materials by neutron diffraction. Journal of Physics: Condensed Matter 20: 244109. doi: 10.1088/0953-8984/20/24/244109
- Kawasaki M et al. (1999) Ionic conductivity of Agx(GeSe3)1−x (0≤x≤0.571) glasses. Solid State Ionics 123: 259-269.
doi: 10.1016/S0167-2738(99)00117-4
- Kozicki MN, Park M, Mitkova M (2005) A low power non-volatile switching element based on copper-tungsten oxide solid electrolyte. IEEE Transactions on Nanotechnology 4: 331-338. doi: 10.1109/TNANO.2005.846936
- Piarristeguy AA, Cuello GJ, Yot PG, Ribes M, and Pradel A (2008) Neutron thermodiffraction study of the crystallization of Ag-Ge-Se glasses: Evidence of a new phase. Journal of Physics: Condensed Matter 20 (15): 155106. doi: 10.1088/0953-8984/20/15/155106
- Ureña MA et al. (2005) Ionic conductivity (Ag+) in AgGeSe glasses. Solid State Ionics 176: 505-512.
doi: 10.1016/j.ssi.2004.09.008
Web References
w1 – To learn more about ILL, see: www.ill.fr
Resources
- Cicognani G (2006) Defying the laws of physics? Science in School 1: 19-21.
- Cicognani G, Capellas M (2007) Silken, stretchy and stronger than steel! Science in School 4: 15-17.
- Hughes D (2007) Taking the stress out of engineering. Science in School 5: 61-65.
- Zaccai G (2009) The intracellular environment: not so muddy waters. Science in School 13: 19-23.
Institutions
Review
This article will be of interest to all who use and appreciate state-of-the-art technologies; the development of programmable metallisation cells illustrates the collaborative elements of nanotechnologies, chemistry, physics and materials science. It also shows the transition from research to commercial potential.
- The article could be used as a comprehension exercise. Possible questions include:
- Outline one advantage and one disadvantage of flash memory devices.
- “For them to function effectively, the important feature of PMCs is …the central solid electrolyte”. Explain the term ‘electrolyte’.
- An integral component in PMCs is a glass. What is a ‘glass’?
- What do you understand by the term ‘neutron diffraction’?
- Much of the activity and construction of these cells is at the nanoscale. What is the nanoscale?
Marie Walsh, Ireland