Mercury: a poisonous solution Understand article
Sigrid Griet Eeckhout from the European Synchrotron Radiation Facility in Grenoble, France, investigates what determines the toxicity of mercury compounds – and how X-ray light is helping to solve the mystery.
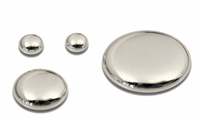
Image courtesy of Mark Evans/
iStockphoto
Metals comprise about 75% of known elements. They can form alloys with each other and with non-metals and are widely used, for example, in cars, computers, highways and bridges. Civilisation was founded upon the metals of antiquity, namely gold, silver, copper, mercury, tin, iron and lead. Gold was first discovered around 6000 BC and mercury has been found in tombs dating back to 1600 BC. The ancient Greeks used mercury in ointments; the Romans used it in cosmetics. Since the beginning of the Industrial Age, metals have slowly entered into the environment, accumulating in soils, sediments and surface waters.
Small quantities of many trace metal elements are of ecological interest due to their necessity as nutrients or their toxicity as pollutants. Nutrient trace elements include magnesium, manganese, copper and zinc, some of which become toxic at high concentrations. Others, including the heavy elements such as mercury, cadmium, arsenic and lead, are of environmental concern due to their high toxicity and widespread industrial use. Mercury is present in the environment at concentrations of less than 0.1%, but is extremely toxic because it binds to the functional groups of various enzymes and proteins, and so inhibits or negatively affects key organic functions. Mercury is used to extract gold, and is found in thermometers, dental amalgam, thermostats, relays, switches, barometers, vacuum gauges and other scientific apparatus, although concerns about its toxicity have led to mercury thermometers being largely phased out in clinical environments.
Mercury is a trace element with both natural (native metal, Hg, and cinnabar, HgS) and anthropogenic (man-made) sources. Anthropogenic sources include agricultural (fungicides) and metallurgic (mining and smelting) uses, plastics industries, refuse disposal and landfills. Most of the mercury in soils, sediments and surface waters comes from the combustion of fossil fuels. This volatile metal can travel over long distances in its gaseous form or by attaching itself to small dust particles. Gaseous mercury can remain in the atmosphere for up to one year before being deposited onto the earth via rainfall.
Once deposited, metals and metalloids (elements with properties of both metals and non-metals) undergo dynamic biogeochemical processes in the near-surface environment, which is a mixture of rocks, soil, water, air and living organisms.
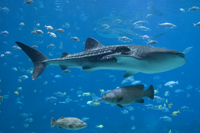
Image courtesy of Klaas Lingbeek-
van Kranen / iStockphoto
Biogeochemical processes affect the metal’s atomic form (speciation), and therefore its solubility, mobility, bioavailability and toxicity. As a rule, the less soluble a chemical species is, the less mobile and less toxic it is. Therefore, transforming soluble species to sparingly soluble forms, either in situ or in landfills after excavation, can lessen the impact of hazardous heavy metals on living organisms and the environment.
Micro-organisms can transform metals by means of oxidation-reduction or other chemical reactions. One example is another heavy metal, hexavalent chromium, Cr(VI), which is a very dangerous, water-soluble form of chromium. Ingesting large amounts of Cr(VI) can cause stomach upsets and ulcers, convulsions, kidney and liver damage, various forms of cancer and even death. Trivalent chromium, or Cr(III), on the other hand, is an essential trace nutrient that helps the body to use sugar, protein and fat. Cr(III) is insoluble in water. Reducing Cr(VI) to Cr(III) using micro-organisms makes it insoluble in water, hence limiting its availability and toxicityw1.
This type of transformation can also occur the other way around. In soils, microscopic organisms can transform the less poisonous, inorganic (non-carbon-containing) form of mercury into a poisonous, organic (carbon-containing) form. In this reaction, called methylation, an atom, usually hydrogen, is replaced by a methyl group (-CH3). As a positively charged ion, methylmercury (CH3Hg+) readily combines with anions such as chloride (Cl–), hydroxide (OH-) or nitrate (NO3–).
Transforming mercury to a methylmercury compound produces a metal form that becomes lipophilic (i.e. can be dissolved in fat) and can thus pass through cell membranes, the blood-brain barrier and placenta. In this organic form it can enter the food chain and accumulate in fish, fish-eating animals and humans. In other words, the less poisonous, inorganic form of mercury, which would normally be safely excreted by organisms, is transformed into an organic form, which becomes available and poisonous to organisms.
So how does the less poisonous, inorganic form of mercury transform into a poisonous, organic form? Researchers from Sweden and the USA used synchrotron light at the European Synchrotron Radiation Facility (ESRF) to determine the speciation of mercury in natural organic matter at environmentally relevant concentrations, using X-ray absorption spectroscopy (XAS) techniques (see box).
They found that mercury in natural soil organic matter binds to two reduced organic sulphur groups, mainly thiols (-SH). The thiol group is the sulphur equivalent of the hydroxyl group (-OH) found in alcohols. Laboratory experiments indicate that neutral, inorganic mercury-thiol and mercury-sulphur species in solution determine the rates of methylation. This means that the binding of mercury to thiol groups in natural organic matter makes the element available for the methylating bacteria in the environment. Furthermore, since these mercury-thiol complexes are soluble, they can be mobilised and transported to places where methylating bacteria live.
The next step is to identify the role that different sulphur-containing molecules frequently found in soil organic matter play in the transformation of mercury to its poisonous form.
The use of XAS to characterise the speciation of mercury is quite new. It is a big step forward compared with earlier wet (liquid phase) biochemical methods and it is the first time that such low concentrations of mercury (0.1 gram of mercury per 1000 grams of soil) have been measured.
Deciphering the chemistry of trace metals and metalloids in the environment is difficult because natural materials are complex in composition and structure. With the advent of advanced synchrotron light sources, which provide techniques using intense X-rays and a greater spatial resolution, scientists are able to determine the forms and distributions of metals in heterogeneous systems such as soils, plants and mineral-microbe-metal interactions. To do this, three micro-analytical techniques can be applied together. The micro-X-ray fluorescence technique (see box) can map the distributions of the different metals and help identify metal associations (Figure 3). Then, the species hosting the metal (such as clay or mineral) is determined at points of interest on the chemical maps by micro-X-ray diffraction and micro-XAS. The diffraction pattern shows the inner structure of the material. The proportion of each species in the bulk material is further calculated by linear combination of the different component species (in other words, by adding up the amounts on the spectra).
In conclusion, X-ray techniques using synchrotron light are extremely valuable in determining the forms and distributions of metals in soils, sediments and surface waters. Once we know the speciation of the metal, we can look into how to limit its solubility and bioavailability. As the world’s population and economies continue to grow, especially in developing countries, the need for metals will increase but their use will amplify the potential for soil and water contamination. Since this could have serious implications for human health and environmental quality, environmental studies are extremely important.
X-ray techniques (for advanced readers)
For a simple explanation of these two techniques, see Capellas (2007).
X-ray absorption spectroscopy (XAS)
X-rays are light with energies ranging from ~500 electron volts (eV) to 500 keV (1 keV is equivalent to 1000 eV).
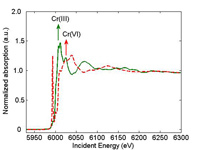
spectrum of a Cr(III) sample
(green) and Cr(VI) sample (red).
The arrow shows the position
of the absorption edge. au =
arbitrary unit.
Click on image to enlarge
Image courtesy of Sigrid Griet
Eeckhout
When you perform an X-ray absorption spectroscopy (XAS) measurement, you vary the energy of the incident X-rays. When the energy of the incident X-ray equals the binding energy of a core-level electron (usually 1s electron), the electron is ejected from the atom. The corresponding X-ray absorption spectrum shows a sharp rise, also known as the absorption edge (Figure 1). The position of the absorption edge is also determined by the formal oxidation state. For instance, the absorption edge of Cr(VI) occurs at higher energy than the one for Cr(III). The outgoing electron interacts with the surrounding atoms, thus creating oscillations in the spectrum beyond the edge. These oscillations provide information on the neighbouring atoms.
Since every atom has core-level electrons with well-defined characteristic binding energies, the XAS technique is element-specific. This means that you can study a chosen element (e.g., mercury) inside heterogeneous matter, such as a soil consisting of organic matter, microbes, minerals, metals and so forth.
The XAS spectrum is sensitive to the formal oxidation state (which reflects the number of electrons available for binding to other atoms), the co-ordination chemistry (e.g., octahedral or tetrahedral co-ordination), and the distances, co-ordination number and species of the atoms immediately surrounding the selected element.
X-ray fluorescence
The well-defined characteristic binding energies of an element are used as a fingerprint in the X-ray fluorescence technique. When you change the energy and you observe a peak at a particular energy, you know that the respective element is present (Figure 2).
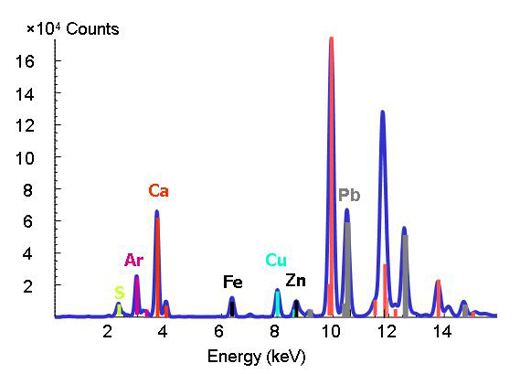
Image courtesy of ESRF
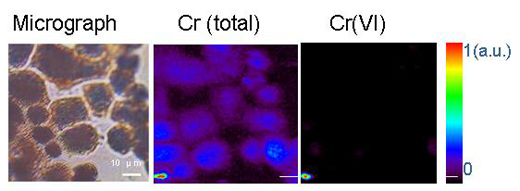
Reprinted with permission from Chemical Research in Toxicology. Copyright (2005) American Chemical Society
References
- Capellas M (2007) Recovering Pompeii. Science in School 6: 14-19. Recovering Pompeii
- Skyllberg U, Bloom PR, Qian J, Lin CM, Bleam WF (2006) Complexation of mercury(II) in soil organic matter: EXAFS evidence for linear two-coordination with reduced sulfur groups. Environmental Science & Technology 40: 4174-4180
- This paper was chosen from more than 1100 articles in Environmental Science and Technology as the top environmental science contribution from 2006.
Web References
- w1 – For a discussion of chromium with reference to the film Erin Brockovich, see:
- Stevens J (2007) Erin Brockovich. Science in School 4: 67-69.
Resources
- A short explanation of the use of synchrotron light at ESRF is available here
- For information about ESRF, see: www.esrf.eu